The Periodic Table
By Tyler Housel, Contributing Editor | TLT Lubrication Fundamentals March 2025
A simplified explanation of chemistry can help us understand the “why” and “how” of atomic interactions.
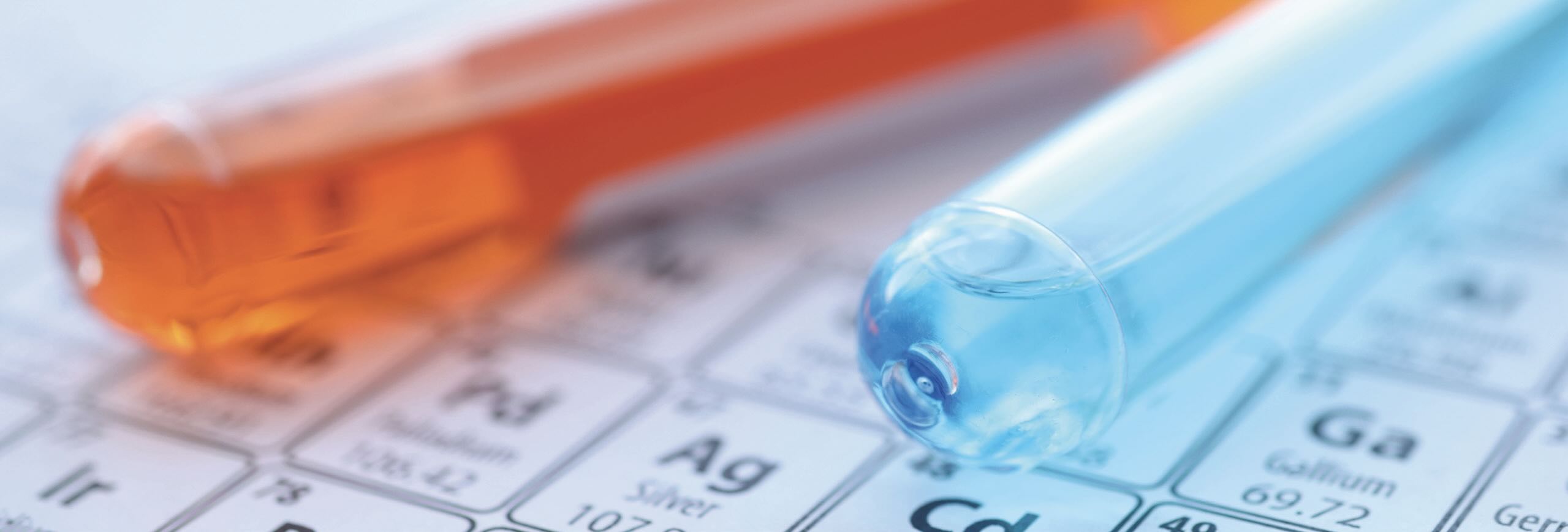
Last month, this column discussed atomism with the assertion that tribologists can create an effective model of the material world based on the atoms in the Periodic Table and their interactions. The aim of this month’s article is to build up an informal structure that explains enough chemistry to answer some of the “why” questions about how atomic interactions manifest into the world we experience. It is impossible to cover the depth and breadth of chemistry in a four-page spread without some oversimplifications. Academic readers can surely find exceptions; under some conditions, xenon can react and the workings of zinc dithiophosphate (ZDDP) are still a mystery. But these simple rules apply in most everyday situations and that makes the exceptions more interesting.
Also, the article uses an anthropocentric approach to describe atomic behavior. Although atoms are not actually “happy” and do not “want” to “take” or “give away” electrons, this language is a useful shorthand way to describe how atoms react as the system “works” toward its most energetically favorable state.
It is easy to get intimidated by the 100+ different elements shown in the Periodic Table. Tribologists can simply ignore many of them. Some elements are so rare that there are fewer atoms on Earth than the number of researchers studying them. Others are heavier analogs of elements with familiar properties. The Periodic Table is more than just a cool image with fancy colors for a t-shirt. Chemists know that the construction of the table and the accompanying colors present a roadmap to understand how different atoms behave. By understanding maybe a dozen important elements, we can use the trends shown in Table 1 to make educated guesses about others.
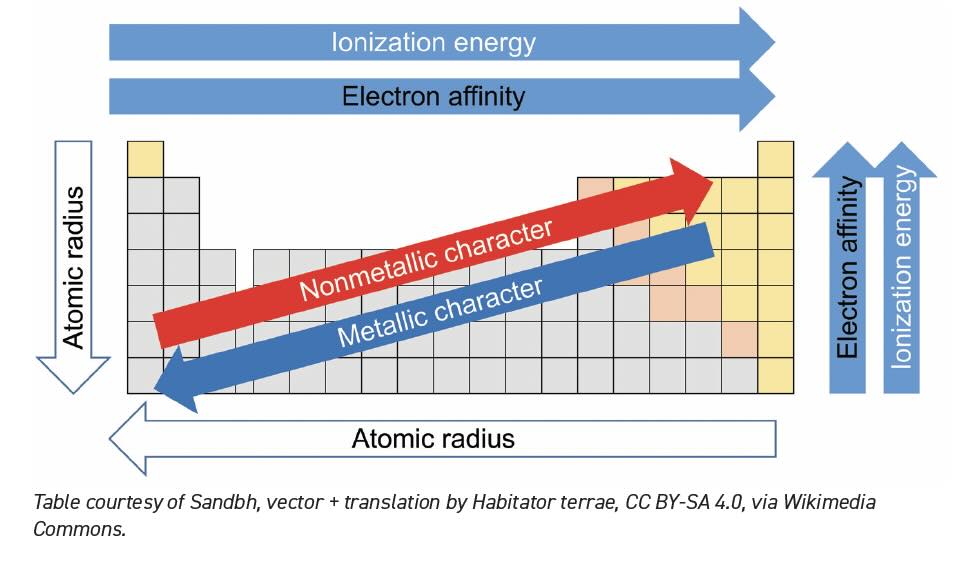
Table 1. Periodic Table Trends.
When the table was conceived by Dmitri Mendeleev1 in the 1860s, he conveniently lined up the known elements in rows (now they are in columns), so the top element has chemical properties similar to the elements below. The first member of the series tends to be its purest representative. The heavier elements in the series exhibit similar chemical behavior but to a lesser degree. It is very impressive that Mendeleev constructed the table from 19th century observational data before many elements were discovered and when atoms themselves were not universally accepted.2 The periodic behavior is easy to explain with our modern understanding of electrons, orbitals and quantum theory, but none of this was known when Mendeleev developed the original Periodic Table.
Atoms are the smallest stable bits of matter on Earth. They have an integral number (1, 2, 3, etc.) of protons in the nucleus, which is identical to their total positive charge (+1, +2, +3, etc.). All atoms of a given element have the same number of protons which are equal to the atomic number. Neutrons are neutral parts of the nucleus that contribute to the atomic weight but do not significantly affect chemistry. Elements can have more than one isotope, so it is important to remember that chemical properties depend on the atomic number rather than the atomic weight (see Table 2).
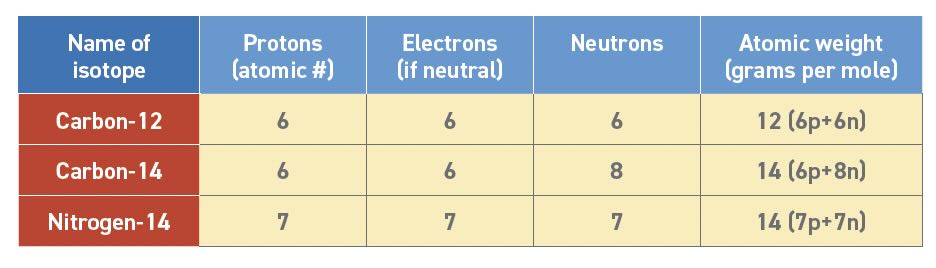
Table 2. Elements of atoms
Electrons are lightweight particles that zip around the nucleus. Each electron has a negative charge precisely equal and opposite to the charge of the proton, so a neutral atom has the same number of electrons and protons.
One of the strange things about electrons is that they can only occupy distinct shells of increasing size and higher energy. The first three shells hold 2, 8 and 18 electrons,3 which correspond to the number of elements in rows of the Periodic Table. After reading several books on quantum mechanics, the best way to explain why is “just trust me.”
Electrons populate the lowest energy shells first and the most stable configurations have all electrons in filled shells. If there are remaining electrons that do not completely fill the next shell, the atom can give, take or share leftover (valence) electrons with neighbors to complete their shells. Essentially, chemistry is a game of gin rummy played between atoms as they swap electrons to fill shells and discard leftovers. The rules are not difficult; the complexity comes from the number of different players.
Table 3 shows the lowest energy electron configurations for elements up to sodium (atomic number 11). Helium and neon have stable configurations, so they are happy and do not trade electrons. Elements like lithium and sodium have one more electron than they need, so they usually push away the extra electron and retreat to the lower filled shell. In Table 1, lower ionization energy shows that electrons are easier to discard. Meanwhile fluorine is just one electron away from a full shell so it will pull an electron from whatever atoms are nearby. The terms electronegativity or electron affinity are used in Table 1 to describe an atom’s tendency to attract valence electrons. The atoms between lithium and fluorine are more than one electron away from a full shell and may make multiple trades to get to their lowest energy ideal state. These trades are chemical bonds.
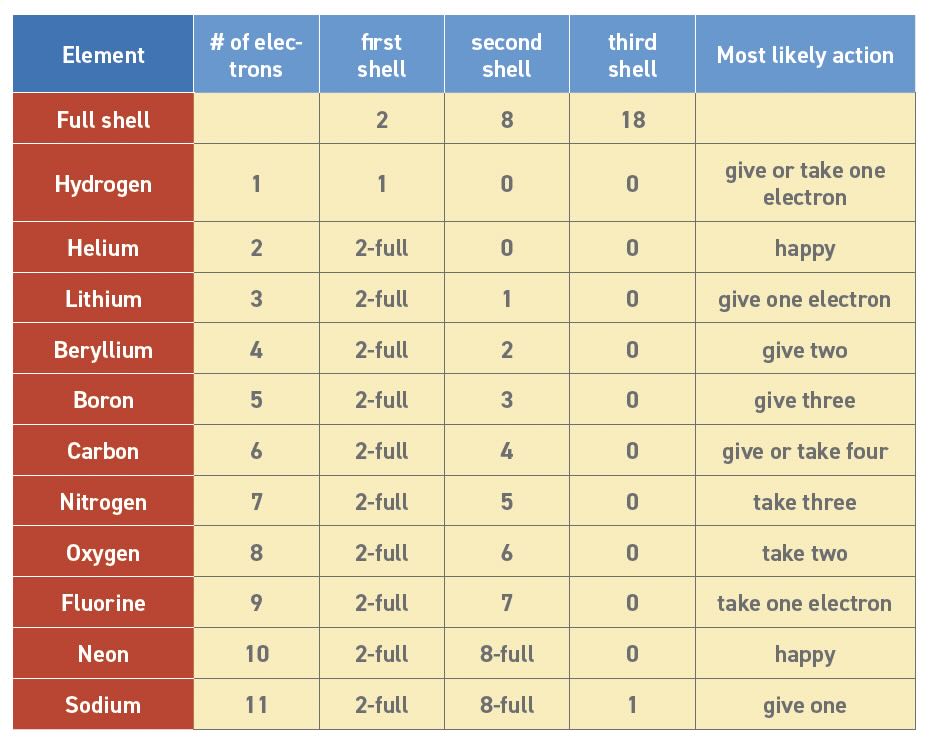
Table 3. Lowest energy electron configurations
Here are some other observations about Table 3:
1. Elements with the same “most likely action” are in the same column of the Periodic Table.
2. The most stable elements have either 2 or 10 electrons and are on the right side.
3. Other elements will give, take or share valence electrons to get to 2 or 10 electron configurations.
4. Carbon has very interesting chemistry because it can make many possible trades.
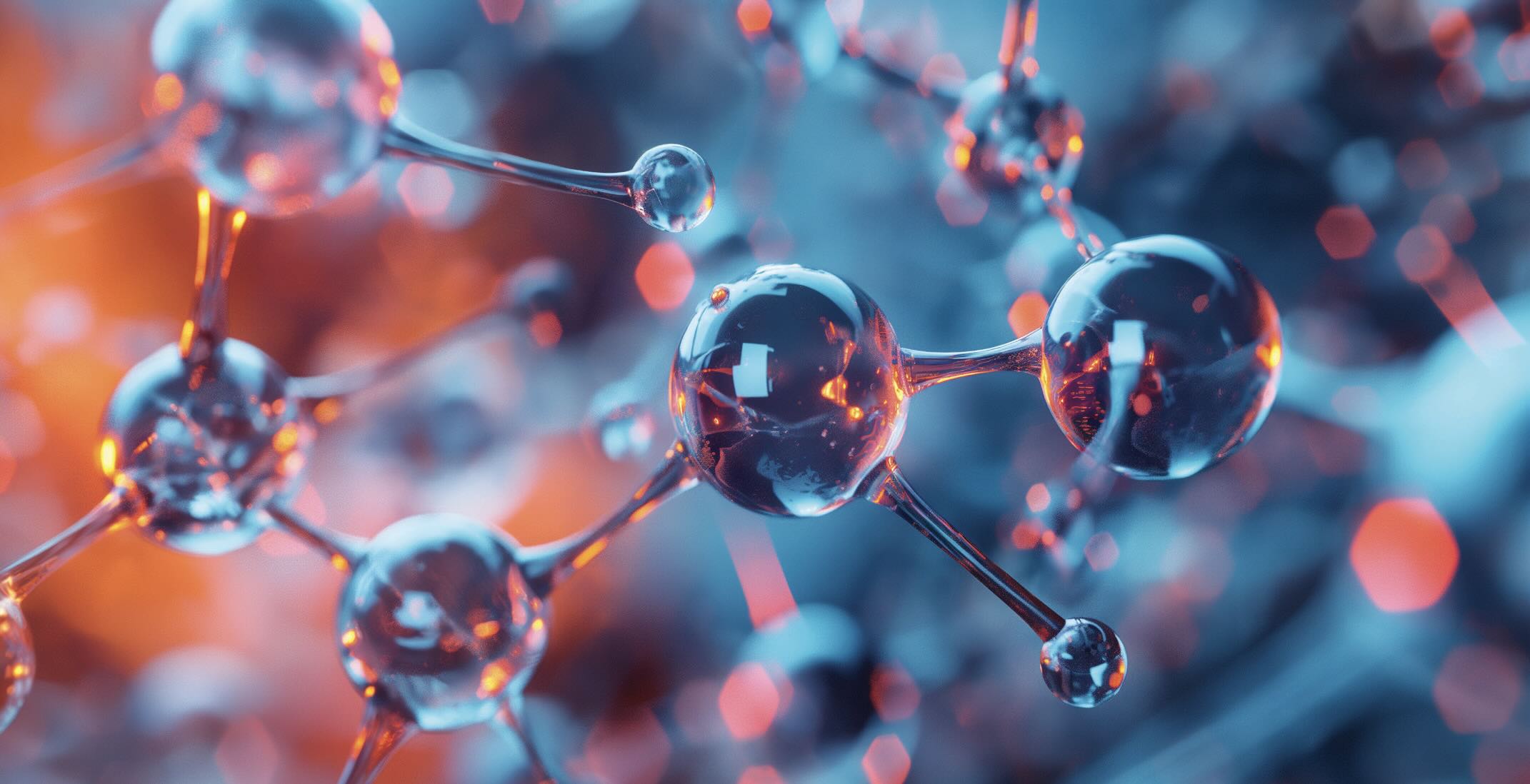
Chemical bonds
An (oversimplified) atomic model (see Figure 1) provides an easy way to picture the interaction between any two atoms without invoking too much quantum mechanics. Each atom has a heavy, positively charged core consisting of the nucleus and inner electrons. Spinning around the core are the light, negatively charged valence electrons. Since the positive core of one atom attracts the electrons from the other, there is an attractive force that strengthens as the cores get closer. However, the two cores repel each other very strongly if they get too close. The electrons are mobile but are prohibited4 from spending all their time between the cores as they approach each other. The electrons move to stabilize the system at a point where the overall bonding energy is minimized (see Figure 2). This nominal separation distance is also known as the bond length. The depth of the attraction curve is strongly influenced by the potential interactions between valence electrons, so bond energy is highly dependent on the atomic pairing. Gravity also contributes a tiny attractive force between atoms that can be ignored.
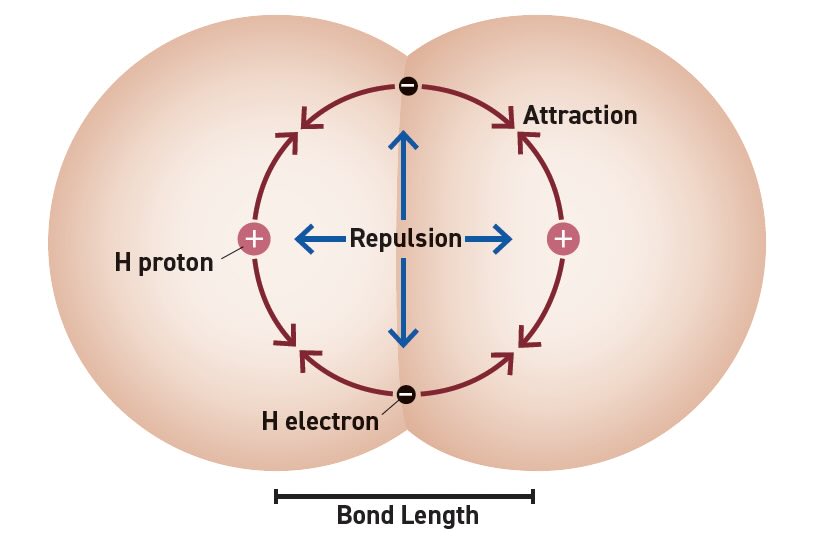
Figure 1. An atomic model.
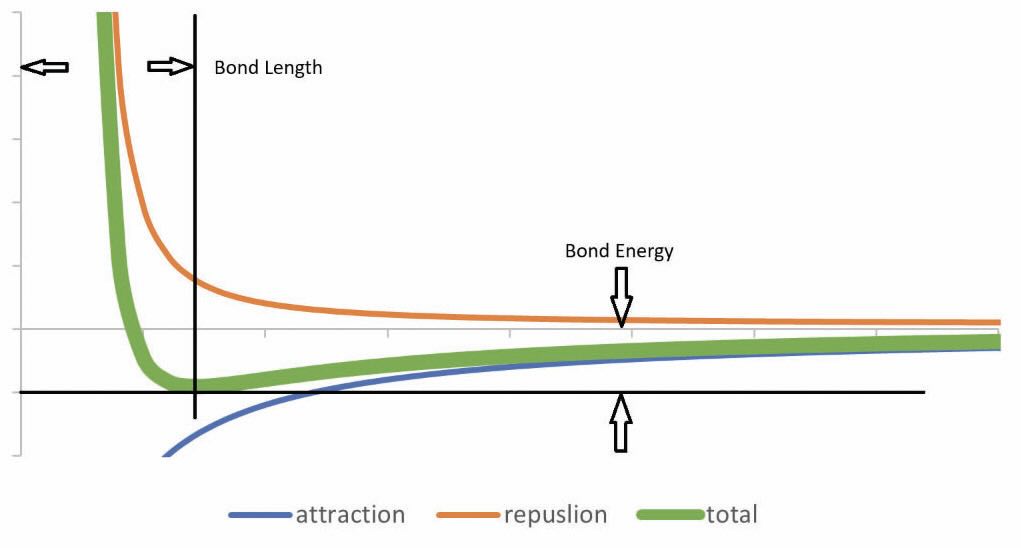
Figure 2. Two atom interaction energy versus separation.
Noble gas atoms
Ignoring kinetic energy, all atom pairs should theoretically bond. But elements with naturally filled shells do not trade electrons so they are not strongly attracted to other atoms and cannot participate in chemical reactions. Noble atoms exist as monatomic gases under ambient conditions because their bond energy is too weak to hold them together. Even at -268°C, helium has enough thermal energy to resist bonding. The noble gas elements are found in the far right column of the Periodic Table and include helium (from the sun), neon (mainly found in Las Vegas), argon (discovered at Argonne National Laboratory), krypton (brought to Earth by Superman) and xenon (used in light bulbs).5
Covalent bonds
As the name implies, covalent bonds are formed when two atoms share valence electrons. Covalent bonds always involve just two specific atoms and once formed they often require a lot of energy to break. Atoms will only share electrons if both want to pull in additional electrons to complete their orbital shell. If they prefer to give away a few electrons and drop to a lower full shell, they will form the metallic or ionic bonds discussed later. Covalent bonding elements are nonmetals located near the noble gases on the upper right side of the Periodic Table because these elements are a few electrons short of a full orbital shell.
Table 2 shows that fluorine atoms need just one electron to have a full second shell with 8 electrons. We know that fluorine forms a stable diatomic molecule (F2) and explain the stability as a covalent bond. Each atom shares one electron with the other and the two shared electrons become a covalent bond. Although the two atoms each start with 7 valence electrons, they form a molecule in which each atom gains the stability of a full shell with 8 total electrons. Covalent bonds are nature’s way of making 7+7=16, by allowing each atom to count the shared electrons as their own. Electron sharing can be confusing so Table 4 provides two examples that may help.
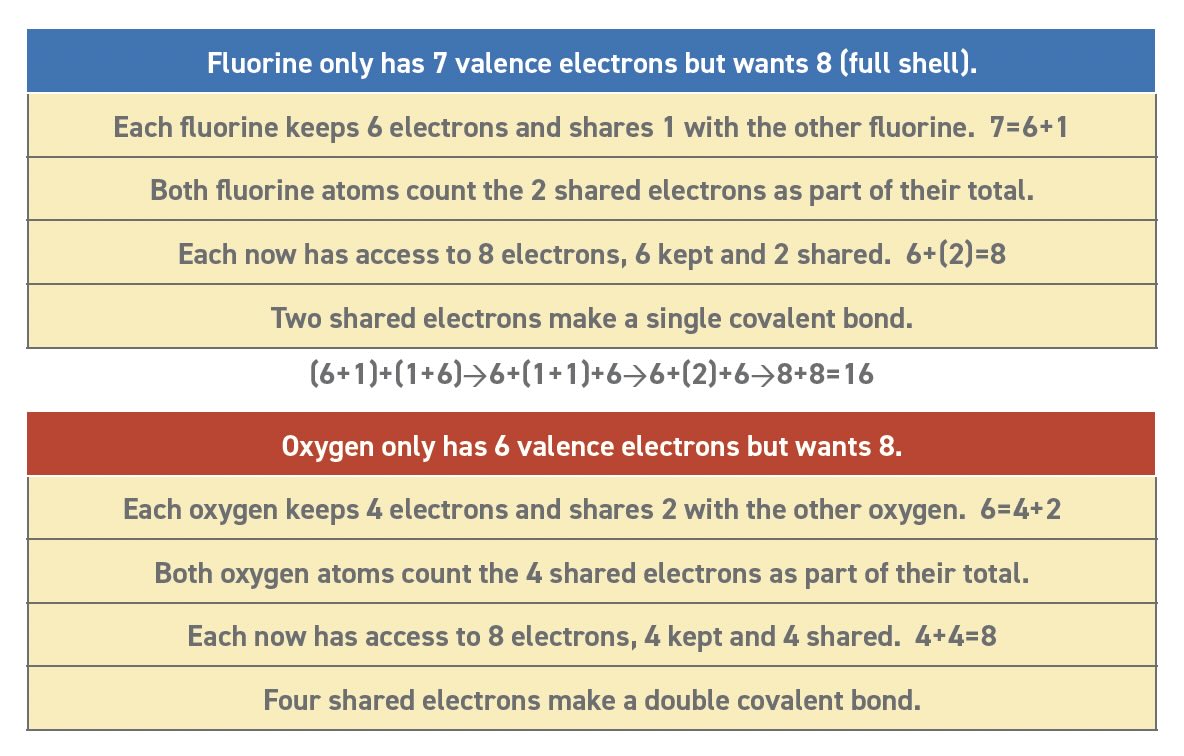
Table 4. Electron sharing
Metals
Elements found in the left, center and most of the bottom parts of the Periodic Table are all metals because they have a few more electrons than they need to fill their core orbital shells. Lithium is the smallest metal atom with just 3 electrons (see Table 2). Two electrons fill the first shell, and the third electron must go into the second shell where there are 8 open positions. The extra electron is not tightly bound because lithium doesn’t have a good place to put it. When many lithium atoms are brought together, these loose electrons are mobile and can easily move from atom to atom. The same concept applies to all metals—they conduct electricity because they have mobile electrons.
Metal oxides
I once heard someone say they avoid plastics and prefer natural materials like metal and glass for food contact. Metal is everywhere nowadays, but the last time someone found a piece of metal naturally occurring on Earth it started the gold rush in 1849. Metals are thermodynamically unstable in Earth’s oxygen atmosphere. Oxygen readily attacks metals for their loose electrons so metal atoms are naturally bound up in metal oxides.
It takes a tremendous amount of energy to produce metals from ore (oxides). Humans invest that energy because metal is useful in our civilization and STLE. But rust never sleeps. Soon after a clean metal surface is formed, atmospheric oxygen begins to oxidize the surface. You can bet that an oxide layer is already forming on the red-hot steel shown in Figure 3. If left unchecked, oxidation can eventually destroy the structural integrity of metal parts. STLE members have developed many strategies to protect metals from oxidation, including alloying, galvanizing, anti-rust and anti-corrosion additives and protective coatings.
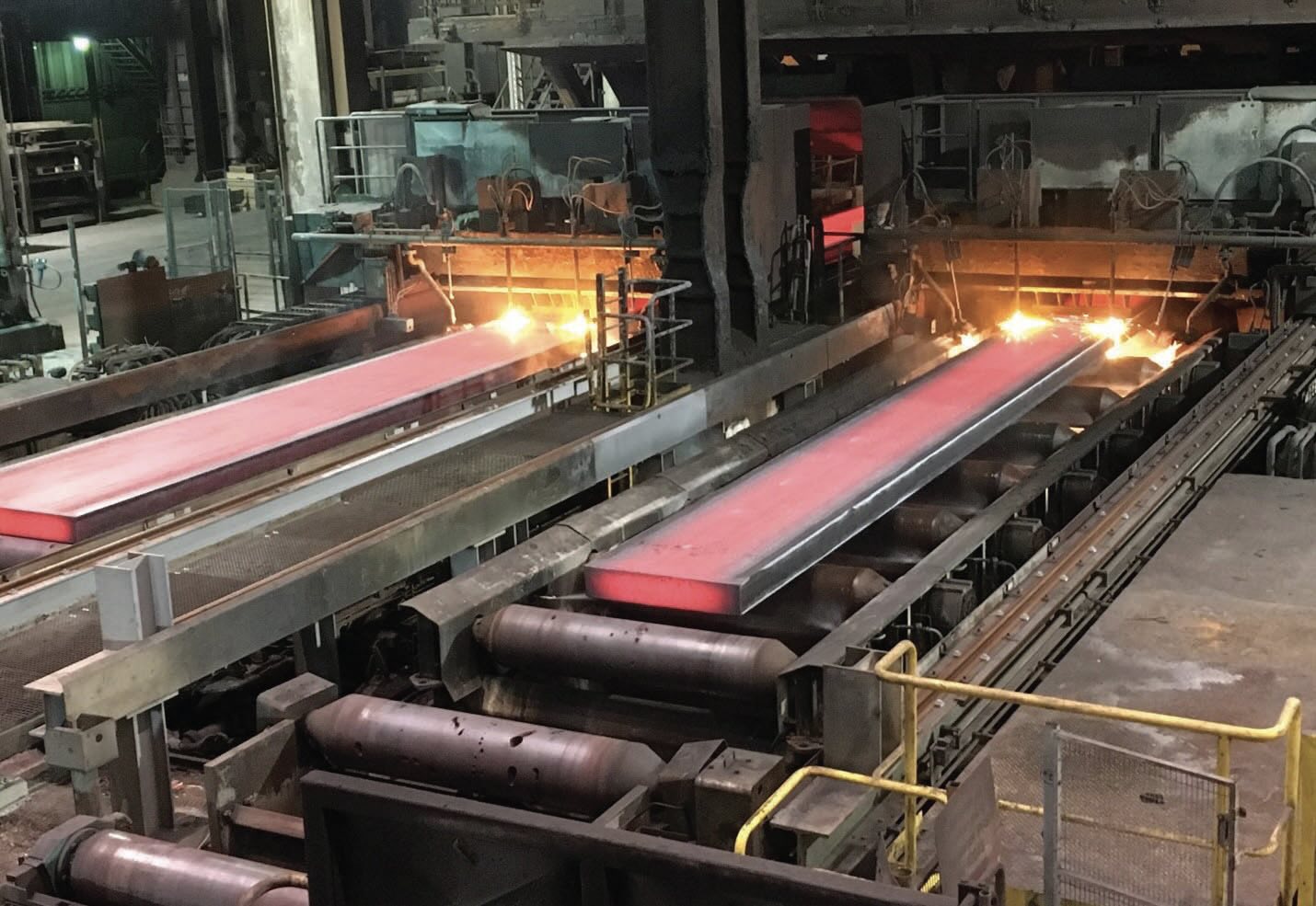
Figure 3. An oxide layer is already forming on red-hot steel.
Ionic bonds
Alkali metals (Li, Na, K, Rb) have one extra electron and halogens (F, Cl, Br, I) are one short of a full shell. When alkali metals and halogens bond, the extra electron is freely given to the halogen (not shared). The term ion refers to any atom (or group of atoms) that gains or loses an electron and carries a net charge. Salts like NaCl form a regular three-dimensional structure of alternating positive Na+ ions (cations) and negative Cl- ions (anions) held together by strong electronic attraction. Pure salt crystals are hard and brittle with a high melting point and low vapor pressure. But most salts readily dissociate in water because polar water molecules stabilize the ions in solution. At the same time, the ions stabilize liquid water, lowering the melting point. This is why salt melts ice.
Although most salts are useless in mechanical systems, ionic liquids are made from large anions and cations that are bulky enough to resist crystallization and therefore remain liquid under ambient conditions. Researchers like STLE member Dr. Ashlie Martini at the University of California, Merced study how the anions and cations can be tuned to create novel, water stable lubricants. The ionic charges help ionic liquids adhere to metal surfaces, forming an effective tribofilm that minimizes friction and wear.
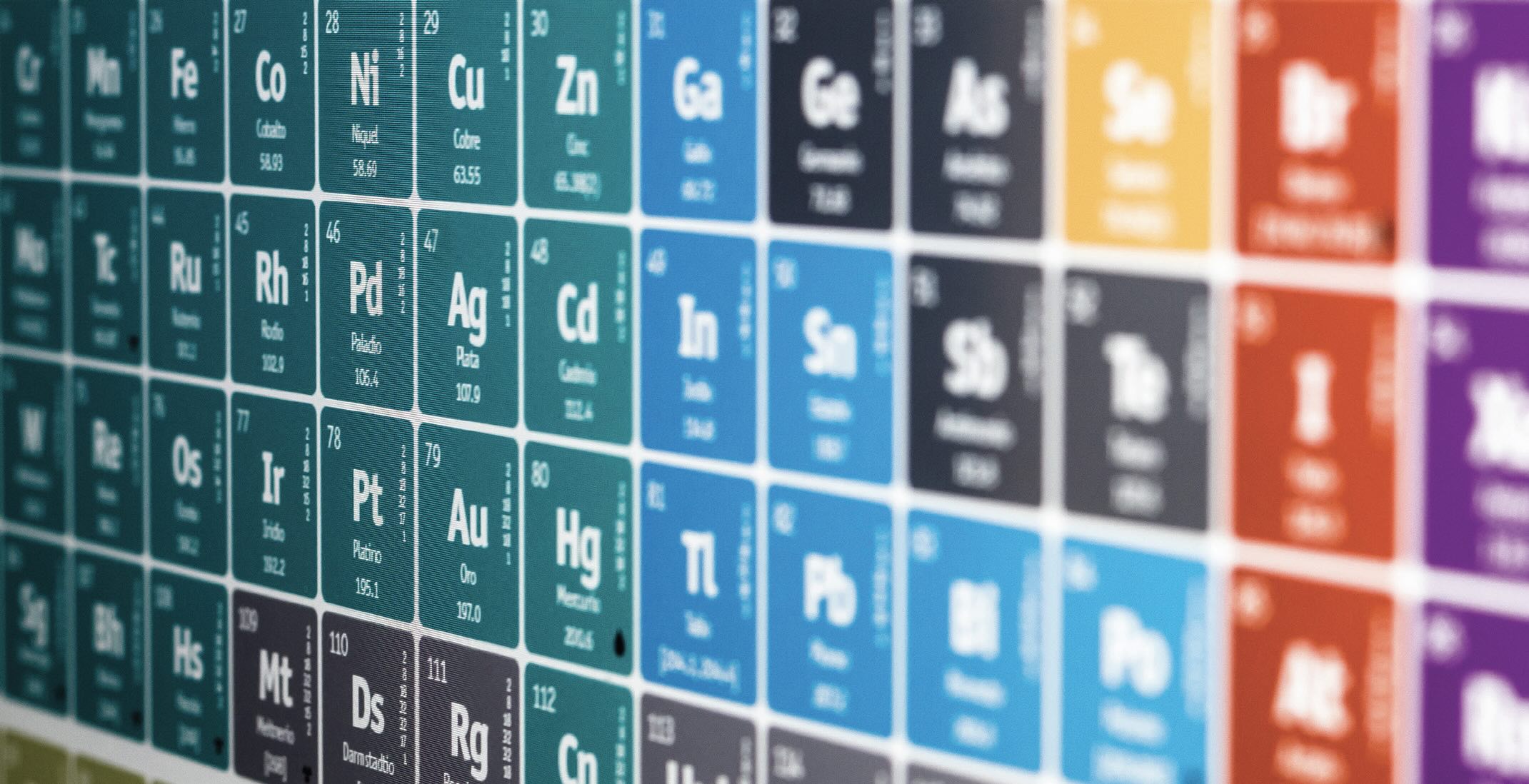
Summary
All two-atom systems can form a chemical bond but will only do so if their bonding energy outweighs the thermal energy. This article describes how bonding energy (bond strength) can be vastly different depending on the electronic compatibility between the pair. The Periodic Table is a chemist’s best tool to understand why some atoms are gases and others combine into nonmetals, metals, oxides and salts. Next month’s article will expand the situation to three dimensions and consider how these rules can even suggest why some interfaces are slippery and others are sticky. After all, that’s what lubrication is all about.
REFERENCES
1. www.britannica.com/biography/Dmitri-Mendeleev
2. Housel, T. (2025), “Atomism,” TLT, 81 (2), pp. 22-27. Available at www.stle.org/files/TLTArchives/2025/02_February/Lubrication_Fundamentals.aspx.
3. There are even bigger shells for elements at the lowest rows of the Periodic Table.
4. Elements in the lower rows of the Periodic Table have even larger shells.
5. I did warn that some of the details in this article were not strictly true.
Tyler Housel is a technologist for Hnuco Technologies and is based in Lansdale, Pa. You can reach him at tylerhousel@comcast.net.