Establishing links between storage environmental conditions, annealing parameters and water mobility in molybdenum disulfide coatings
By Nicolas Molina and Filippo Mangolini | TLT Fellowship Research July 2024
Texas Materials Institute, The University of Texas at Austin, Austin, Texas 78712, United States
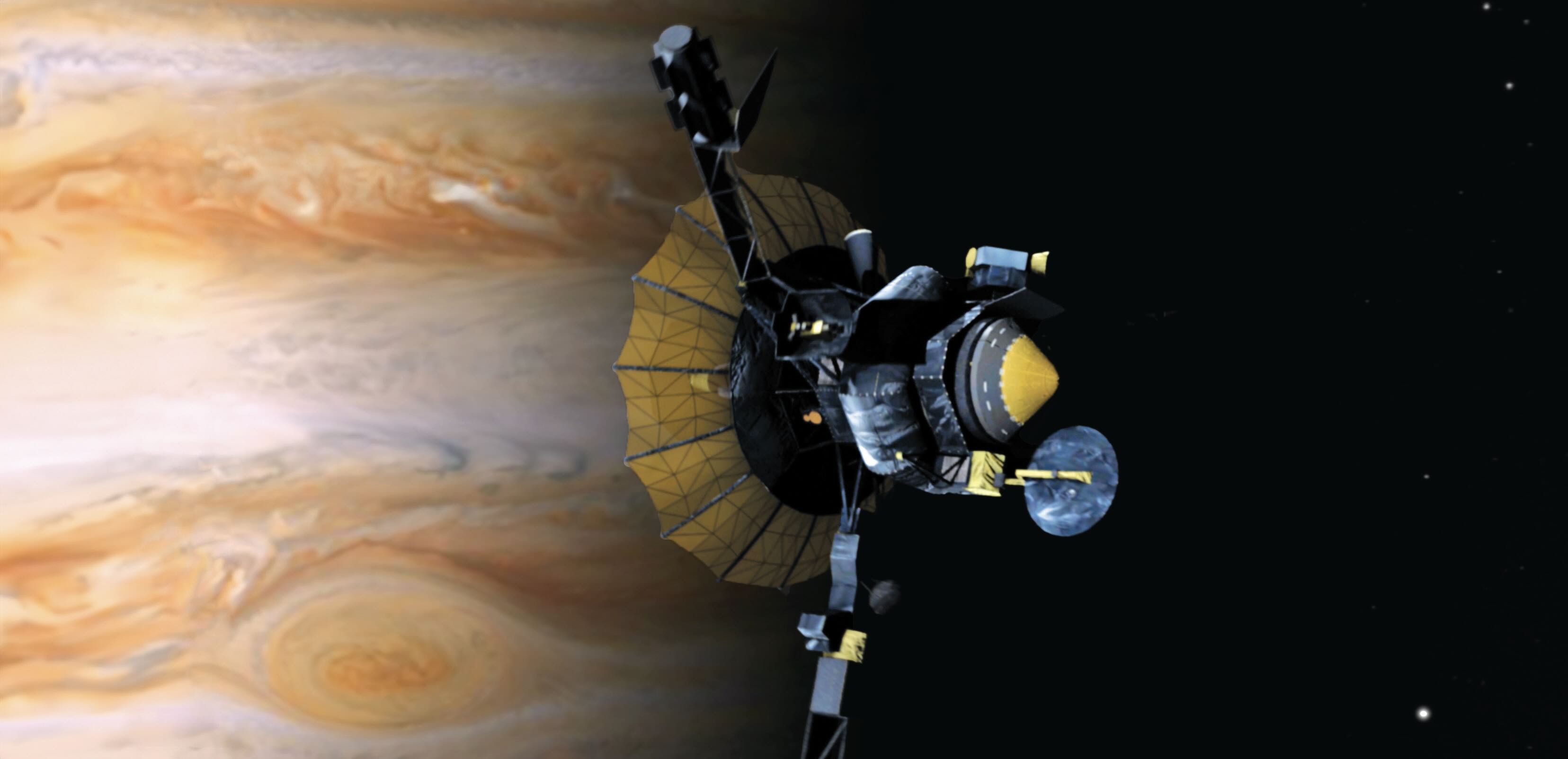
Editor’s Note: This month TLT profiles the 2023 recipient of The Elmer E. Klaus Fellowship, Nicolas Molina Vergara (University of Texas at Austin). The Klaus Fellowship, along with The E. Richard Booser Scholarship, are awarded annually to graduate and undergraduate students, respectively, who have an interest in pursuing a career in tribology. As a requirement for receiving an STLE scholarship, students are given the opportunity to participate in a tribology research project and to submit a report summarizing their research. For more information on the Elmer E. Klaus Fellowship, visit www.stle.org.
Nicolas Molina obtained his master of science degree in mechanical and metallurgical engineering from the Pontific University of Chile, being awarded three tribology-oriented research internships during his studies in France, Poland and the U.S. He is currently a doctoral student in materials science and engineering at the University of Texas at Austin working under the supervision of STLE member Dr. Filippo Mangolini. His doctorate research focuses on the spectroscopic evaluation of the surface and bulk chemical processes occurring in solid lubricants upon tribological testing and aging under different environmental conditions. Molina can be reached at namolina@utexas.edu.
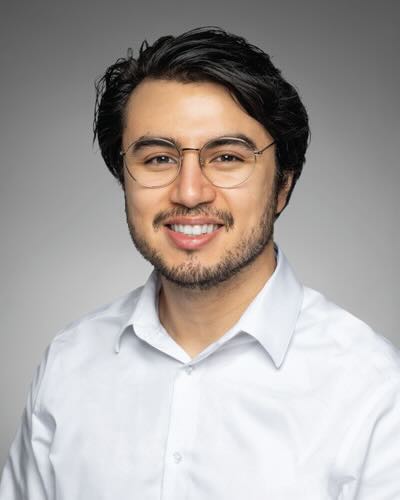
Nicolas Molina
Abstract
Molybdenum disulfide (MoS2) coatings are often used in tribological components working under inert and vacuum conditions due to their low steady-state coefficient of friction. However, prolonged periods of storage and dormancy of these components allow time for the diffusion of surface adsorbates, such as water, into the coating, modifying the overall friction behavior when sliding is resumed (i.e., aging effect). Despite the relevance of published studies on the surface chemical processes underpinning aging of MoS2 coatings, our understanding of the depth distribution of water within MoS2 thin films remains elusive. In this work, a depth-dependent, chemistry-informed study of the water mobility in non-contact area and wear track of MoS2 coatings aged in humid conditions and under various annealing treatments was performed. The depth distribution of water (OH—) and MoS2 (92MoOx— and 92MoSx—) ion fragments were traced by time-of-flight secondary ion mass spectrometry (ToF-SIMS). The results reveal the significant influence of both the chemistry of the storage environment and annealing conditions on the mobility of water in MoS2. Altogether, this work provides valuable insights for the underlying mechanisms leading to variations in friction performance of MoS2 thin films across nanoscale and macroscale applications upon storage under different conditions.
1. Introduction
Molybdenum disulfide (MoS2) is a lamellar solid that has been extensively used in space applications owing to its low coefficient of friction (μ < 0.05) and wear rates (< 1 x 10–6 mm3 N–1 m–1) in inert or vacuum environments[1–3]. The frictional behavior of MoS2 is characterized by a transition from a higher initial coefficient of friction (μ > 0.1) to a low steady-state coefficient of friction (μ < 0.05) upon sliding[4]. This run-in process has been attributed to the shear-induced realignment of MoS2 basal planes in the contact area, providing a low shear-strength interface between the tribopair[5] that can be further enhanced by the transfer of MoS2 to the counter body[6].
The impact of the working and storage conditions (from high vacuum to low vacuum, and from dry to humid conditions) of MoS2-coated components on their frictional behavior has been extensively evaluated[7, 8]. For example, when sliding in an inert environment (O2 < 0.5 ppm, H2O < 0.5 ppm) is resumed after long periods of dormancy in the same environment, the previously reached steady-state coefficient of friction is not achieved anymore in the first sliding cycles. Rather, a new run-in characterized by an increase in the coefficient of friction (μ > 0.05) is observed[8]. This phenomenon is referred to as the stop-time, dwell or aging effect, and has been attributed to the interaction of oxygen and water with Mo-edges or vacancies[9–11], as well as the intercalation of water between MoS2 flakes, which affects the van der Waals interactions between MoS2 layers[12].
While, to the best of our knowledge, the aging processes occurring in MoS2 have been studied by evaluating the physical or chemical interactions of oxygen and water with the material[13, 14], little is known about the effect of the chemistry of the storage environment and annealing conditions on the water mobility within sputtered-MoS2 coatings. Gaining insights into the depth distribution of water and simultaneously the distribution of surface contamination and oxides in MoS2 is experimentally challenging owing to the required use of quantitative analytical methods having high surface sensitivity and depth resolution. Among all characterization techniques with sub-nanometer depth resolution, time-of-flight secondary ion mass spectrometry (ToF-SIMS) is ideally suited as it allows for the detection of hydrogen[15] as well as ion cluster fragments from the coating (e.g., MoSx— and MoOx—), thus enabling the identification of bonding interactions between several species within the probed region[13, 16]. In the case of water diffusion in MoS2 thin films, ToF-SIMS would thus allow tracing water by sputtering a surface and collecting the characteristic fragments of this molecule.
In this work, we evaluate the depth distributions of molecular ion fragments corresponding to water (OH—) and MoS2 (92MoOx— and 92MoSx—) and correlate them with various treatment conditions for the coatings, with a focus on pre-annealing. The outcomes of this study provide insights into the effects of pre- and post-treatments on MoS2, providing a basis for understanding and optimizing their tribological performance after storage for prolonged periods of time.
2. Materials and Methods
2.1. Sample preparation and tribological testing
Molybdenum disulfide (MoS2) coatings were prepared at Sandia National Laboratories (Albuquerque, N.M.) via direct current (DC) magnetron sputtering under 10 mTorr pressure of argon gas. The substrates were 1 cm × 1 cm n-type Si wafers <100> (Ra < 1 nm). High-purity MoS2 and Ti sputtering targets (Angstrom Sciences, Inc.) were used to respectively deposit 650-nm-thick MoS2 films at 200 W bias voltage and a 75-nm-thick Ti adhesion layer at 300 W bias voltage.
After deposition, the samples were tribologically tested via reciprocating ball-on-flat test under a dry N2 environment (O2 < 20 ppm, H2O < 50 ppm). The normal load applied to the 1/8" diameter 440C steel ball and the sliding velocity were 200 mN (∼0.6 GPa maximum Hertzian pressure) and 1 mm/s, respectively. After testing, the samples were vacuum sealed and stored in polyethylene specimen bags with silica gel. A humidity indicator card inside the specimen bag was used to monitor the relative humidity, which was less than 10% at all times. The samples were sent to the University of Texas at Austin for further assessment.
2.2. Sample characterization
To evaluate the as-deposited microstructure of the samples, grazing-incidence X-ray diffraction (GIXRD) measurements were carried out on a control MoS2 coating using a Rigaku Ultima IV X-ray diffractometer (Rigaku Corporation, Japan) with Cu Kα radiation (λ = 1.5406 Å) and operating at 40 kV and 44 mA. The spectra were acquired between 5 and 90° (2θ). The penetration depth of the X-ray was estimated using the GIXA tool developed by the X-ray group at the Atominstitut of the Vienna University of Technology (Austria), resulting in ∼1 μm at a fixed incidence angle (ω) of 3.6° (above the total-reflection critical angle), assuring that the adhesion layer and substrate background noise were minimally contrib- uting to the coating X-ray diffraction pattern while attaining a high X-ray yield. The X-ray diffraction pattern of the MoS2 coating showed basal (002) or columnar (110) diffraction peaks, indicating a polycrystalline film structure.
2.3. Experimental technique
To assess the depth distribution of ion cluster fragments (e.g., H2O—, MoS2—, MoO3—), time-of-flight secondary ion mass spectrometry (ToF-SIMS) measurements were carried out using a TOF.SIMS 5 instrument (ION-TOF GmbH, Germany). The instrument is equipped with a pulsed (20 ns) bismuth ion gun (30 keV) and a Cs+ /O2 + sputtering ion gun (0.5/1 keV, 40 nA). All profiles were acquired in high mass resolution mode for the mass analyzer (6,000 m/δm), and spectrometry mode for the bismuth ion gun (3 pA). The profiles were acquired in non-interlaced mode, i.e., sequential analysis (100 µs cycle time, 256 × 256 pixels, i.e., 6.5 s), sputtering (0.5 s), and pause (1 s) to reduce potential charging of the sputtered surface. The analysis was performed with a monoatomic Bi1 + source to enhance the yield of secondary ion fragments, and the sputtering was performed with Cs+ in negative polarity since it promotes the emission of negatively charged secondary ion clusters bonded to hydrogen, oxygen and sulfur. The primary ion beam was randomly raster-scanned over a 100 μm × 100 μm (< 1 × 10–12 ions/cm2), while the sputtering ion beam was centered over the 300 μm × 300 μm analysis area (> 1 × 10–14 ions/cm2). The base pressure of the load lock and analysis chamber was always below 1 × 10–7 Torr and 1 × 10–9 Torr, respectively.
Raw fragment yields as a function of sputter time were processed using the native ION-TOF SurfaceLab7 software (IONTOF GmbH, Germany). The recorded mass range, 0-850 m/z, was calibrated with CH— (13.00 m/z), C2— (24.00 m/z), CHO— (29.00 m/z), C2O (33.90 m/z), CO2 (43.99 m/z), CHO2 (44.99 m/z), C2H3O2 (59.01 m/z), CO3 (59.98 m/z) for the top-surface contamination, and 92MoO— (107.90 m/z), 92MoS— (123.87 m/z), 92MoSO— (139.87 m/z), 92MoS2— (155.85 m/z), 92MoS2O— (171.84 m/z), 92MoS3— (187.82 m/z) for the high mass region. Point-to-point normalization was performed with respect to the total intensity at each sputter time to account for sputtering-induced surface roughening, ionization probability variations due to matrix effects, and changes in the transmission of secondary ions with sputter depth[17]. To ensure consistency of the analysis, the relative sensitivity factors for the ion clusters of interest were not employed due to lack of known values for a MoS2 matrix. The ion clusters of interest were exported for further post-processing in Igor Pro 9.06 (WaveMetrics, OR).
2.4. Experimental procedure
The as-deposited samples were handled inside an MBraun Argon glovebox (MBraun, Germany) with low levels of moisture ([H2O] < 1 ppm) and oxygen ([O2] < 1 ppm) to suppress water uptake and oxidation within the MoS2 coatings[14]. The samples were mounted and arranged at the center of a topmount holder and then transferred to the ToF-SIMS load lock chamber using an air-free transfer vessel.
To evaluate the diffusion of water into sputtered-MoS2 coatings, the following procedure was carried out: First, two samples were transferred to the analysis chamber to evaluate the initial water content of the non-contact area and wear track via ToF-SIMS depth profiling. One sample was then pre-annealed at 150°C for 2 hours at a base pressure of 3 × 10–5 Torr to assess the effect of annealing on the diffusion of water. Next, both samples were aged in humid air (relative humidity between 40% and 50%) for 55 days (1,320 hours) and then evaluated via ToF-SIMS depth profiling. The samples were post-annealed at 150°C for 2 hours at a base pressure of 3 × 10–5 Torr and once again evaluated with ToF-SIMS to assess the water desorption phenomena in the near-surface and bulk of MoS2.
2.5. Data processing
The theoretically possible ion cluster fragments of H2O are H2O—, HO—, H2— and H—. Among these ions, only HO— and H— were experimentally detected by ToF-SIMS. The yield of hydrogen ion fragments (H— or H+) has been shown to vary considerably not only due to the reasons mentioned in the point-to-point normalization procedure (see Section 2.3), but also because of their dependence on the analysis chamber pressure, analysis and sputtering beam and acquisition mode[15]. Furthermore, their secondary ion translational kinetic energy distribution is distinctly different from other monoatomic ion fragments[18]. Consequently, HO— is selected as the tracer fragment of H2O.
The sputter depth calibration for MoS2 was performed by post-mortem evaluation of 20 sputter zones using a Wyko NT 9100 Optical Profilometer (VEECO Instruments, Plainview, N.Y.) in vertical scanning interferometry mode (out-of-plane resolution: 2 nm, maximum z-range 2 mm), and was found to be 0.097 ± 0.005 nm/s. For practical purposes, it was assumed to be constant throughout the sample thickness.
3. Results and Discussion
3.1. ToF-SIMS depth profiling of noncontact area
The depth distributions of molecular ion fragments corresponding to water (OH—) under various treatment conditions are shown in Figure 1 for the non-contact area. Comparative analysis with the as-received specimen reveals notable distinctions in the samples aged in humid air, which are characterized by a depletion of water between 0.3 and 2 nm. Subsequently, a gradual increase in water content toward the bulk is observed until reaching a steady-state regime, occurring at approximately 23 nm for the specimen aged in humid air and 24 nm for the pre-annealed sample that was subsequently aged in humid air. Remarkably, pre-annealing results in reduced water content in the near-surface, while also facilitating a significant increase in water diffusion into the bulk. Both specimens exhibit identical inflection points at ~0.3 nm (#1), ~0.9 nm (#2), ~1.9 nm (#3), ~3.8 nm (#4) and ~6.2 nm (#5), delineating regions with distinct water mobility.
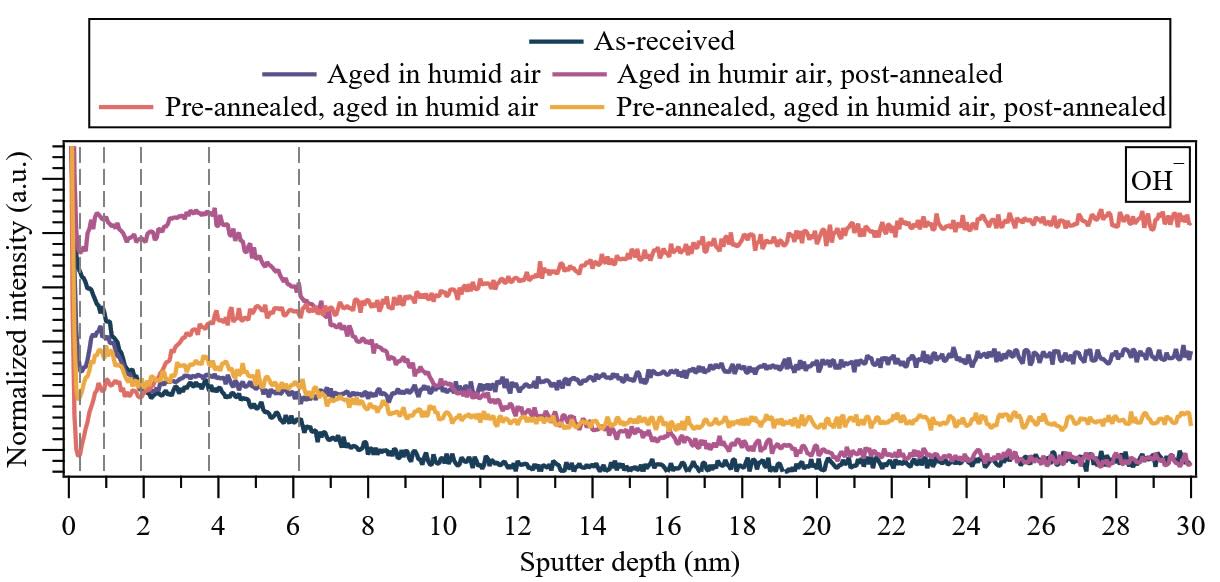
Figure 1. (Non-contact area) Depth distributions of molecular ion fragments corresponding to water (OH—) under various treatment conditions for 650-nm-thick PVD sputtered-MoS2 coatings. Dashed lines highlight inflection points observed in the water depth distributions. Note: The first major tick mark in the y-axis is 40 × 10–3, and the major tick mark increment (Δ) is 5 × 10–3.
Upon post-annealing, a bulk-to-surface diffusion of latent water within the specimen occurs in the non-contact region aged in humid air, which enriches the near-surface water content. Similarly, this phenomenon is observed in the pre-annealed sample aged in humid air and then post-annealed, although with a less pronounced enrichment near the surface. This finding is indicative of a more effective water desorption from the coating in the latter sample. Therefore, pre-annealing is shown to lower the diffusion barrier in the near-surface, facilitating water desorption when the coating undergoes post-annealing (note: the steady-state water level is only comparable to the as-received specimen for the sample aged in humid air without pre-annealing). Notably, the inflection point around 6.2 nm (#5) is absent upon post-annealing, as also observed in the as-received specimen.
To elucidate the enrichment and depletion of water within the non-contact area and discern the origin of inflection points (#1 through #5), the depth distributions of molecular ion fragments corresponding to MoS2 (92MoOx— and 92MoSx—) were investigated (see Figure 2).
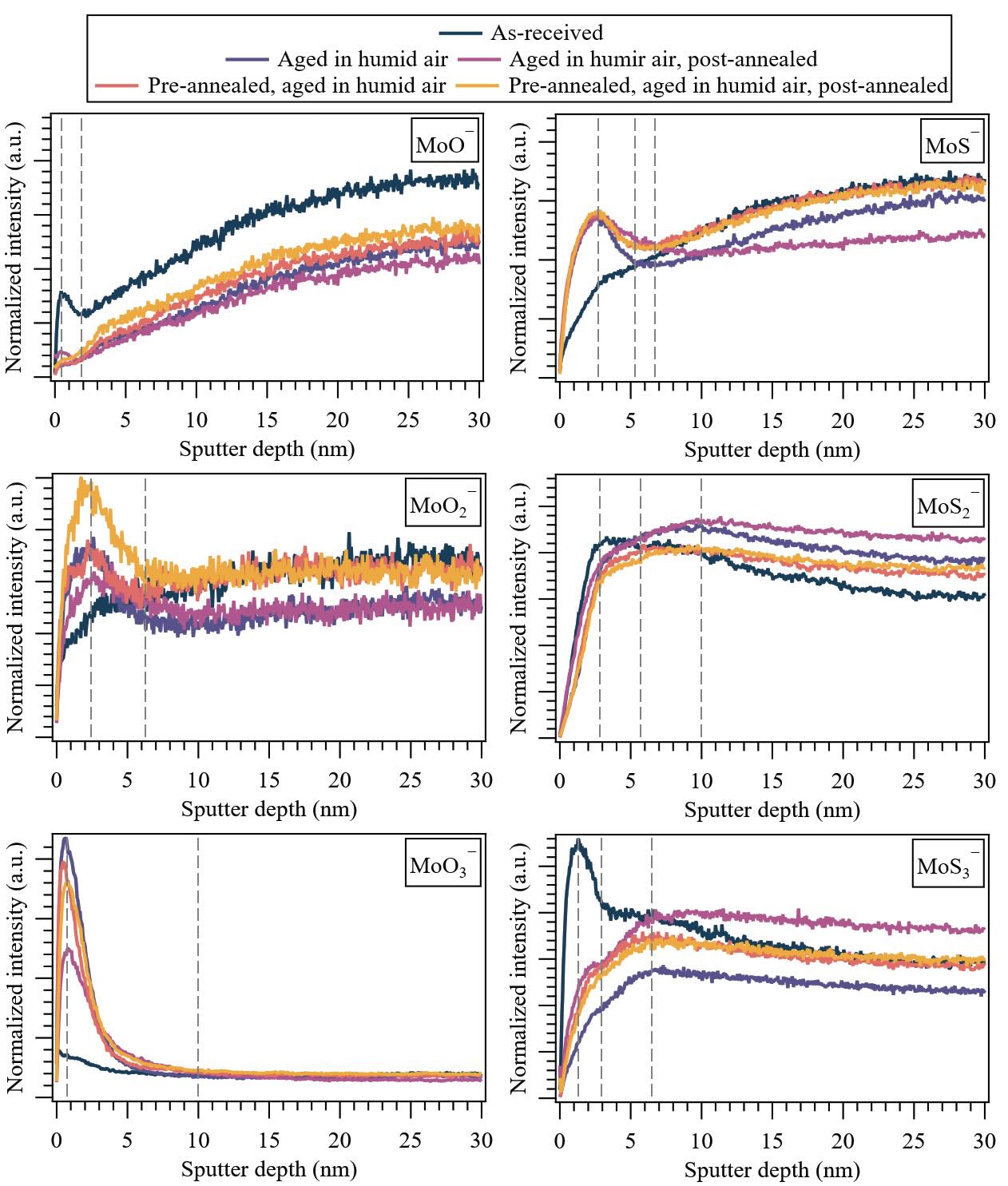
Figure 2. (Non-contact area) Depth distributions of molecular ion fragments corresponding to MoS2 (92MoOx— and 92MoSx—) under various treatment conditions for 650-nm-thick PVD sputtered-MoS2 coatings. Dashed lines highlight general inflection points observed in the MoS2 ion fragment depth distributions. Note: The first major tick mark in the y-axis is 0 for all plots, and the major tick mark increments (Δ × 10–3) are: 0.5 (92MoO—), 0.25 (92MoO2—), 10 (92MoO3—), 2.5 (92MoS—), 5 (92MoS2—) and 1 (92MoS3—).
First, the evolution of the yield of the ion fragments of MoS2 (92MoOx— and 92MoSx—) with depth reveals a gradient in chemistry within the sputtered-MoS2 coating. A significant fraction of molybdenum atoms bonded to oxygen (92MoO—, 92MoO2—, 92MoO3— ion clusters) was found throughout the probed depth. Specifically, 92MoO3— exhibited the highest yield at the top surface (~1 nm), followed by 92MoO2— (~2.5 nm), while 92MoO– progressively increased in intensity with depth until ~28 nm.
No statistically significant difference in the depth profiles of 92MoO3— was observed beyond 10 nm, suggesting that the bulk oxidation of MoS2 is not characterized by heavy ion clusters (92MoOx>2—) but rather by small fragments (92MoOx≤2—). When compared to the as-received specimen, aging in humid air (regardless of pre- or post-annealing) led to increased levels of 92MoO2— and 92MoO3— in the near-surface region, accompanied by a decrease in 92MoO— ion clusters. This indicates the progressive enrichment of the oxidized surface layer of heavy oxides in the non-contact near-surface upon aging. The effect of post-annealing cannot be generalized, as it varies depending on the sample’s treatment. For the sample aged solely in humid air, post-annealing resulted in a decrease in 92MoO2— and 92MoO3— signals in the near-surface region. Conversely, for the pre-annealed sample that was also aged in humid air, post-annealing led to an increase in 92MoO2— signal while slightly decreasing the 92MoO3— signal in the near-surface region. Overall, pre-annealing was found to increase the signals of 99MoO— and 92MoO2— in the bulk.
Consistent with the earlier results, it was observed that the 92MoSx— ion fragments corroborate the gradient of chemistry within the sputtered-MoS2 coating. Specifically, 92MoS3— exhibited the highest intensity at the top surface for the as-deposited sample (~1.5 nm), however, for samples aged in humid air (irrespective of pre- or post-annealing), the maximum intensity was found deeper at ~6.5 nm. This shift in depth correlates to the oxidation of the near-surface region, leading to a reduction in the fraction of molybdenum atoms bonded to sulfur – a process that potentially generates localized domains comprising small molecular fragments, which increase across the surface upon aging (92MoS—).
From a chemical reaction perspective, the presence of oxygen atoms bound to molybdenum originates from the dissociative adsorption of water and oxygen during or following the deposition process, as the simultaneous presence of these molecules leads to the following oxidative reactions[16, 19]:
2MoS2 + 9O2 + 4H2O → 2MoO3 + 4H2SO4 2MoS2 + (9-2x)O2 → 2MoO3 + 4SO(3-x), with x = 0 or 1
The reaction product MoO3 undergoes fragmentation during ToF-SIMS analyses, resulting in the formation of 92MoO—, 92MoO2— and 92MoO3— molecular ion clusters, as detected in the present work. Notably, a rapidly decaying 92MoO3— signal detected in the outermost region of the sample, and 92MoO— and 92MoO2— presence throughout the coating, may indicate the presence of different oxidation states across the coating. This observation agrees well with previously published X-ray photoelectron spectroscopy (XPS) results, which reported that both as-deposited samples and samples aged in humid environments contain a high fraction of molybdenum atoms in oxidation state +4 (Mo(IV), i.e., MoO2), rather than exclusively +6 Mo(VI) (i.e., MoO3)[14].
Altogether, these findings offer further evidence of the evolving oxidation state of molybdenum as a function of depth in sputtered-MoS2 coatings, underscoring the importance of considering the gradient in chemistry within the MoS2 coating when assessing the diffusion and concentration profile of water throughout the coating. The inflection points thus reflect the local abundance of MoOx and MoSx species within the non-contact area, delineating different regions where water diffusion occurs, as summarized in Figure 3.
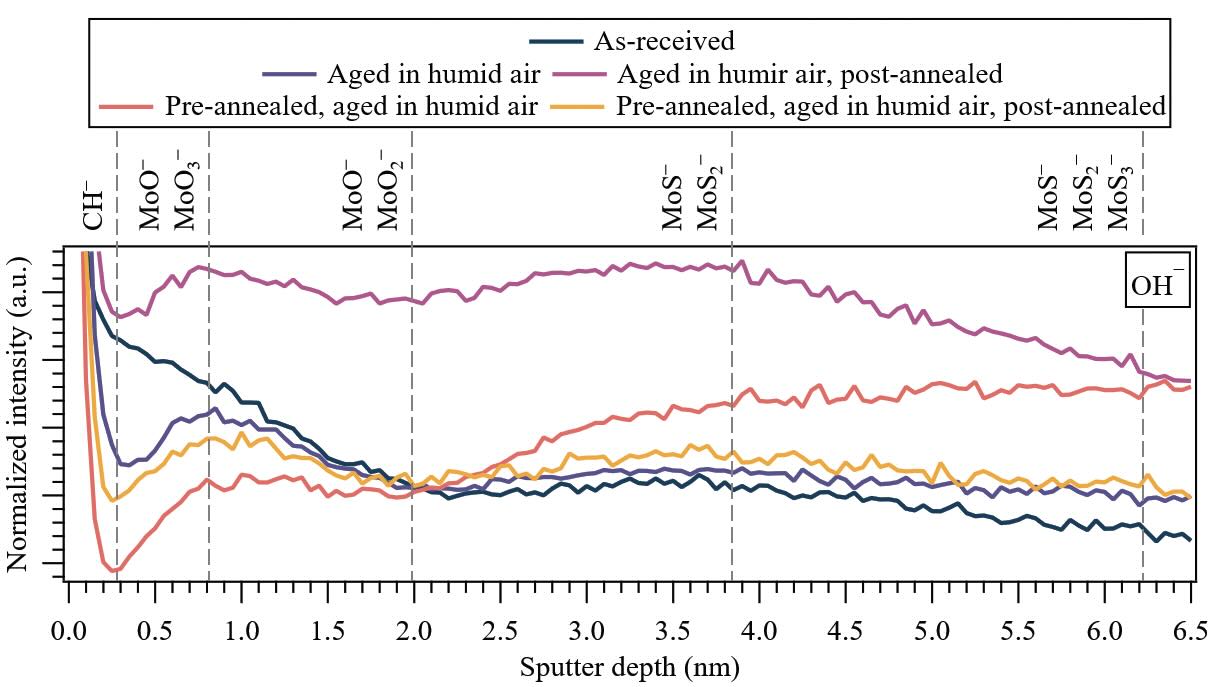
Figure 3. (Non-contact area) Near-surface depth distributions of water (OH—) under various treatment conditions for 650-nm-thick PVD sputtered-MoS2 coatings. The dashed lines indicate the positions where ion fragments corresponding to MoS2 exhibit local/global maxima. Note: The first major tick mark in the y-axis is 40 × 10–3, and the major tick mark increment (Δ) is 5 × 10–3.
The molecular ion fragments corresponding to water (OH—) reveal a distinct region of adsorbed water in the contamination layer (0-0.3 nm) (CH— molecular ion fragment not shown), followed by an increasingly effective diffusion barrier (bulk-to-surface) characterized by a higher presence of oxides (from 92MoO3— to 92MoO2—) up to 2 nm. This is succeeded by a second, slightly less effective diffusion barrier (from 92MoO2— to 92MoS—), and finally a region with reduced water retention characterized by molecular ion fragments corresponding to the coating (92MoS2— to 92MoS3—).
An exception to this sequence is observed in the non-contact area of the sample that was pre-annealed and aged in humid air, where the behavior after the first diffusion barrier involves a continuous increase in water retained within the coating. This can be attributed to a change in the microstructure upon annealing, as reported in[14], where the fraction of (103) and (110) edge planes increases compared to the as-received specimen. According to the Density Functional Theory (DFT) simulations, water dissociation is thermodynamically favorable on Mo-terminated (100) edge planes[10] but likely unfavorable for sulfur-terminated (110) planes of MoS2. Consequently, more water is intercalated between MoS2 lamellae instead of being dissociated[12, 20], leading to an increase in stored water in the bulk.
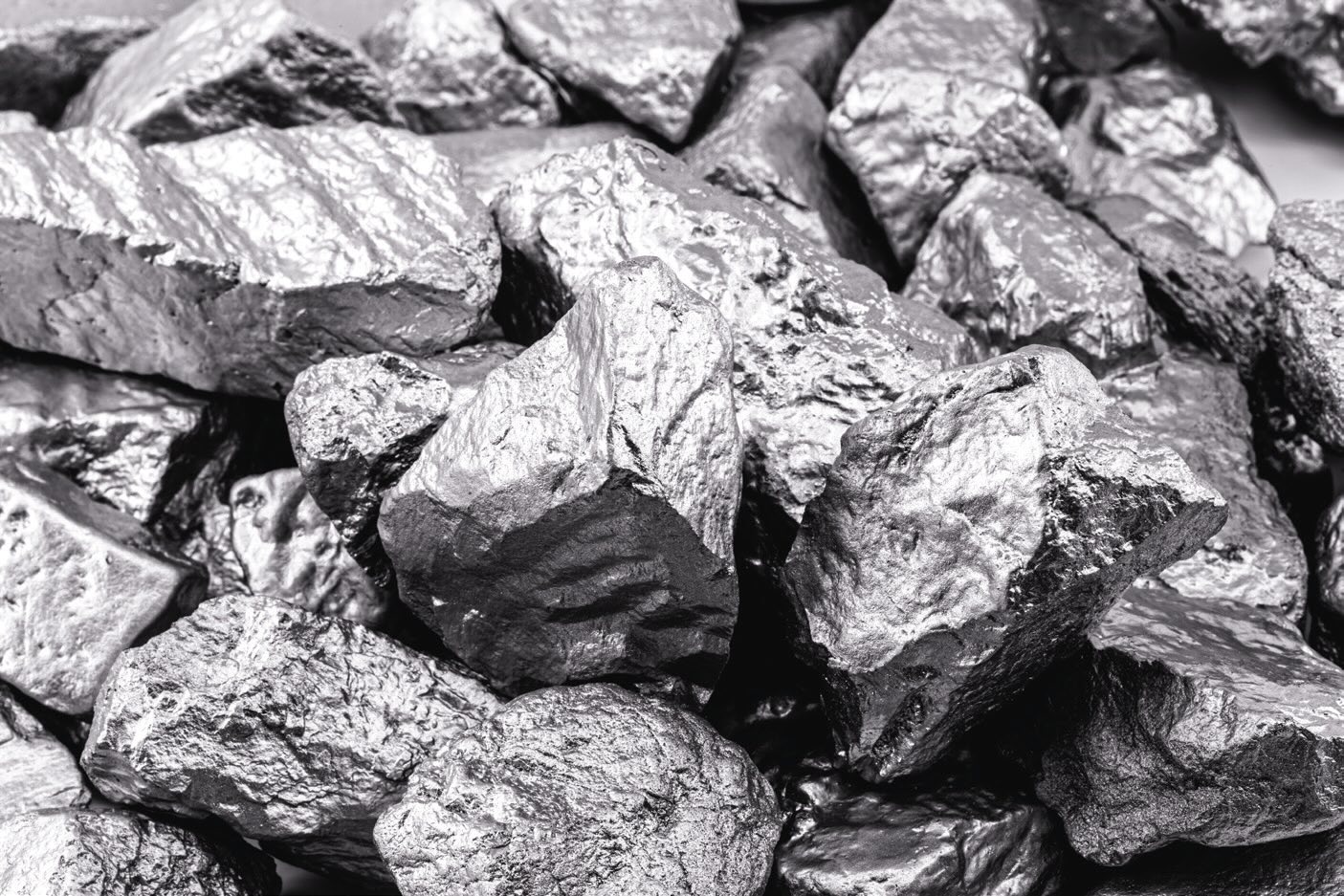
3.2. ToF-SIMS depth profiling of wear track
The depth distributions of molecular ion fragments corresponding to water (OH—) under various treatment conditions are shown in Figure 4 for the wear track. In contrast to the non-contact area, comparative analysis with the as-received specimen reveals significant distinctions among the samples aged in humid air. Specifically, the wear track aged solely in humid air is characterized by a depletion of water between 0.5 and 1 nm, whereas the pre-annealed wear track exhibits an enrichment of water in this region. Moreover, a gradual increase in water content toward the bulk is observed for the wear track aged in humid air until reaching a steady-state regime at approximately 25 nm. In contrast, a concave behavior with significant water sorption is observed for the pre-annealed wear track, reaching a steady-state regime at a deeper depth (~28 nm) compared to the non-contact area (~24 nm). Notably, pre-annealing results in increased water content in the near-surface while facilitating a significant increase in water diffusion into the bulk. Both treatments exhibit different regions with distinct inflection points, with fewer evident inflection points (4) compared to the non-contact area (5).
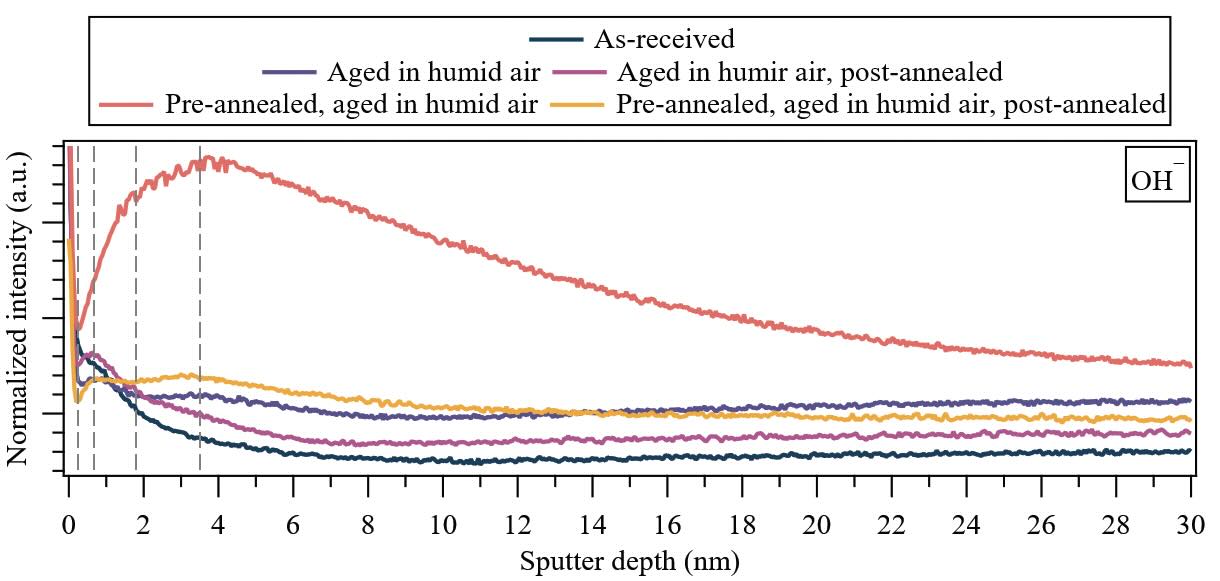
Figure 4. (Wear track) Depth distributions of molecular ion fragments corresponding to water (OH—) under various treatment conditions for 650-nm-thick PVD sputtered-MoS2 coatings. Dashed lines highlight general inflection points observed in the water depth distributions. Note: The first majortick mark in the y-axis is 50 × 10–3, and the major tick mark increment (Δ) is 25 × 10–3 .
Upon post-annealing the wear track aged in humid air, a bulk-to-surface diffusion of latent water within the specimen occurs, slightly enriching the near-surface with water. This is indicative of effective water desorption from the wear track. Similarly, this bulk-to-environment phenomenon is observed in the pre-annealed wear track aged in humid air after post-annealing. However, contrary to the wear track aged in humid air and post-annealed non-contact areas, there is a depletion of water in the near-surface after annealing. Based on these results, we conclude that pre-annealing lowers the diffusion barrier of water in the near-surface, facilitating water desorption when the coating undergoes post-annealing. The steady-state water level of the as-received specimen is not comparable to any treatment. Notably, the inflection point around 3.5 nm (#4) is absent for the wear track aged in humid air and post-annealed, as well as the as-received specimen, indicative of changes in the chemical state or microstructural morphology around that region.
To elucidate the enrichment and depletion of water within the wear track and discern the chemistry behind the inflection points (#1 through #5), the depth distributions of molecular ion fragments corresponding to the coating (92MoOx— and 92MoSx—) were investigated in Figure 5.
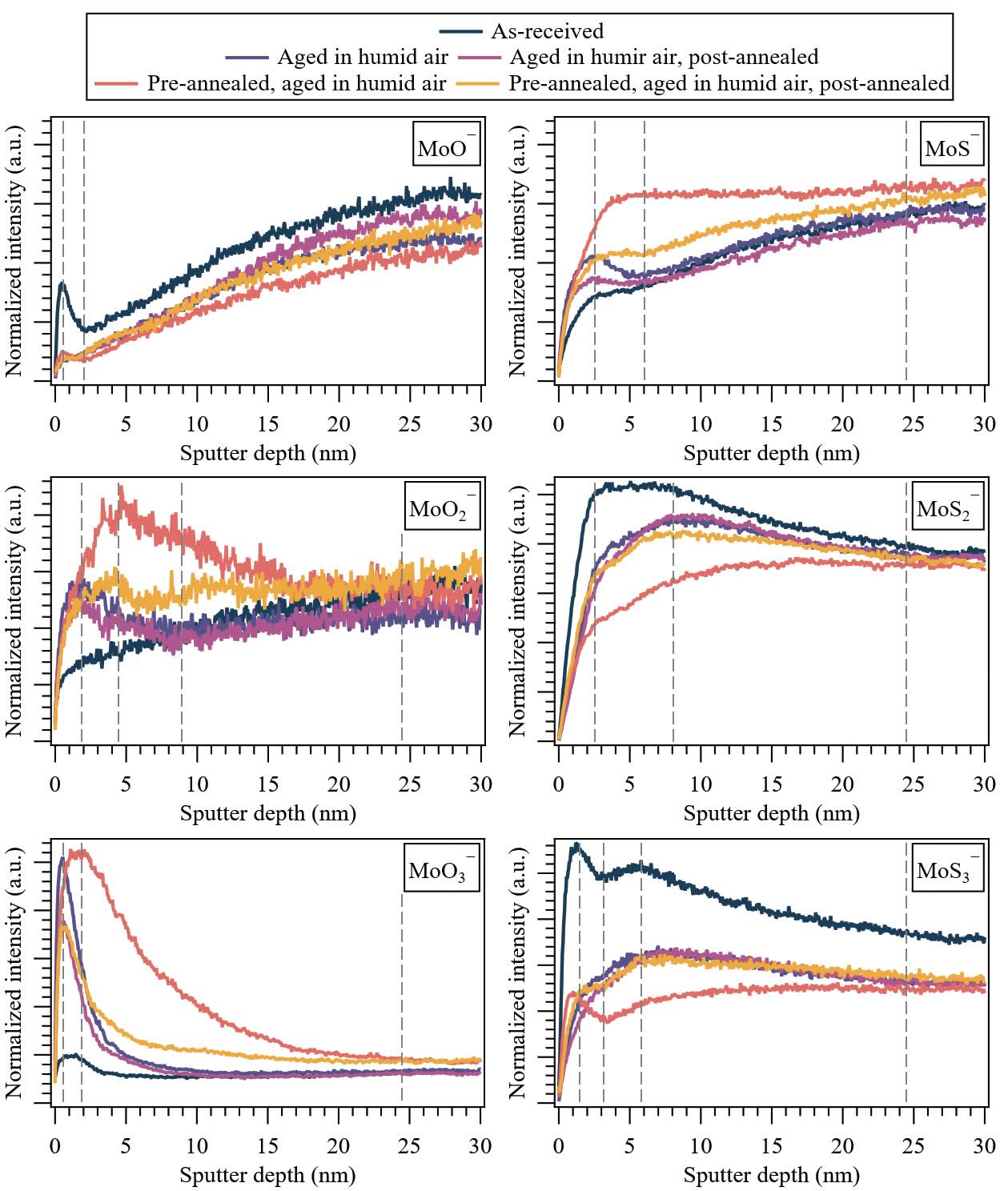
Figure 5. (Wear track) Depth distributions of molecular ion fragments corresponding to MoS2 (92MoOx— and 92MoSx—) under various treatment conditions for 650-nm-thick PVD sputtered-MoS2 coatings. Dashed lines highlight general inflection points observed in the MoS2 ion fragment depth distributions. Note: The first major tick mark in the y-axis is 0 for all plots, and the major tick mark increments (Δ × 10–3) are: 0.5 (92MoO—), 0.25 (92MoO2—), 5 (92MoO3—), 2.5 (92MoS—), 5 (92MoS2—) and 1 (92MoS3—).
Similarly to the non-contact area, the ion fragments of the coating (92MoOx— and 92MoSx—) within the wear track unveil a gradient in surface chemistry. A significant fraction of molybdenum atoms bonded to oxygen (92MoO—, 92MoO2—, 92MoO3— ion clusters) was observed throughout the probed region, exhibiting varying degrees of yield and depth distribution. Specifically, 92MoO3— displayed the highest intensity at the top surface (~0.8 nm), followed by 92MoO2— (~1.8 nm), while 92MoO— exhibited progressively increasing yield with depth until ~28 nm. An exception to the location of these maxima is observed for the pre-annealed wear track aged in humid air, with a deeper maximum location for 92MoO3— (2 nm) and 92MoO2— (4.5 nm), which aligns with the distinct OH— distribution for this treatment (see Figure 4).
Statistically, a difference in the depth profiles of 92MoO3— was detected beyond 10 nm for the pre-annealed wear track, indicating that pre-annealing can promote bulk oxidation of MoS2 subjected to tribological testing. This leads to an increased presence of heavy ion clusters (92MoOx>2—) with broader and higher yields compared to untreated wear tracks. Comparatively, aging in humid air (irrespective of pre- or post-annealing) led to elevated levels of 92MoO2— and 92MoO3— in the near-surface region, accompanied by a decrease in 92MoO— ion clusters. As is the case with the non-contact area, this indicates an increase in heavier oxides in the wear track. In contrast to the non-contact area, the effect of post-annealing was consistent and can be generalized, as it resulted in a decrease in 92MoO2— and 92MoO3—signals in the near-surface region, while slightly increasing the signals of 92MoO— and 92MoO2– in the bulk.
In line with the previous observation, the 92MoSx— molecular ion fragments within the wear track corroborate the gradient in surface chemistry. Specifically, 92MoS3— exhibited the highest yield at the top surface for the as-deposited specimen and pre-annealed/ aged in humid air sample (~1.5 nm), while for other treatment conditions, the maximum yield was found deeper (at 6 nm). As observed in the non-contact area, this shift in depth correlates with the oxidation of the near-surface region, resulting in a reduction in the fraction of molybdenum atoms bonded to sulfur. Interestingly, upon post-annealing, the overall oxidation decreased. This phenomenon can be attributed to a change in the microstructure upon pre-annealing, where the increase in (100) edge planes compared to the as-received wear track, as reported also in[14], makes the wear track favorable for water dissociation, aligning with the significant increase in 92MoO2— and 92MoO3— throughout the coating. However, further annealing (i.e., post-annealing) can potentially lead to the formation and desorption of H2O[21], diffusing back to the surface and decreasing the 92MoO2— and 92MoO3— molecular ion fragments, as observed previously. Hence, the location of inflection points depends on the local abundance of MoOx and MoSx species within the wear track, delineating different regions where water diffusion occurs, as summarized in Figure 6.
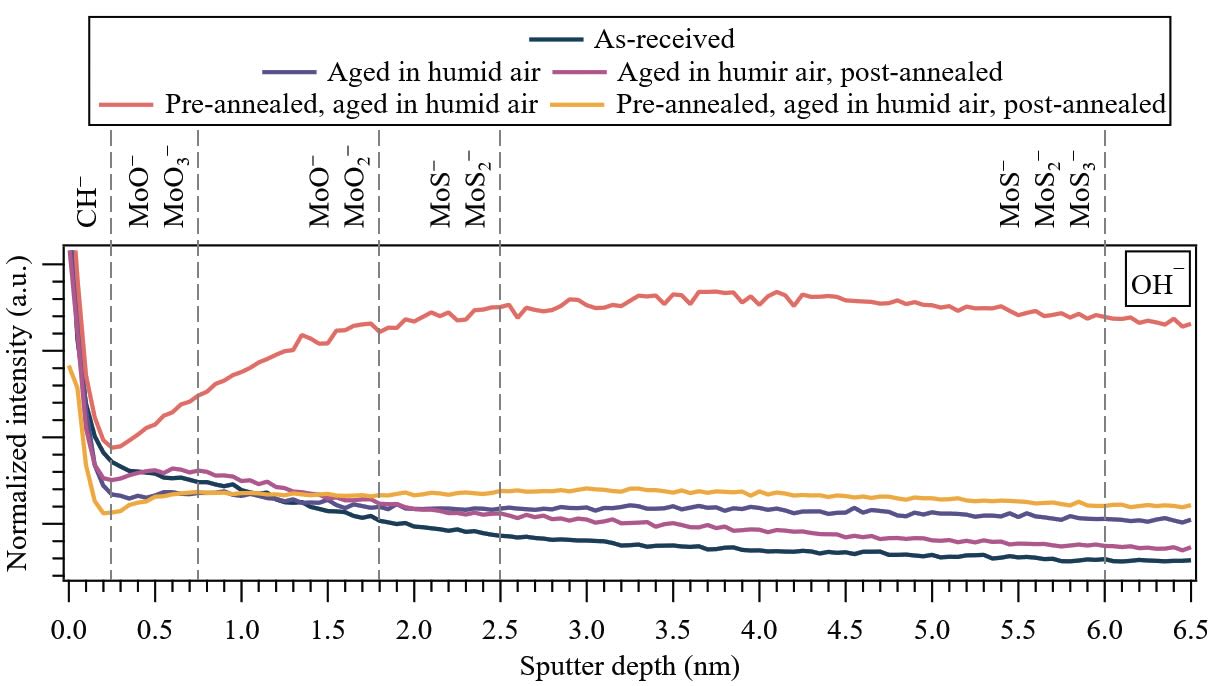
Figure 6. (Wear track) Near-surface depth distributions of water (OH—) under various treatment conditions for 650-nm-thick PVD sputtered-MoS2 coatings. The dashed lines indicate the positions where ion fragments corresponding to MoS2 exhibit local/global maxima. Note: First major tick mark in the y-axis is 50 × 10–3, and major tick mark increment (Δ) is 25 × 10–3.
The molecular ion fragments corresponding to water (OH—) reveal a distinct region of adsorbed water in the contamination layer (0-0.25 nm) (CH— molecular ion fragment not shown), followed by an increasingly effective diffusion barrier (bulk-to-surface) characterized by a higher presence of oxides (from 92MoO3— to 92MoOM2—) up to 1.8 nm. This is succeeded by a second, slightly less effective diffusion barrier (from 92MoO2— to 92MoS—) extending up to 2.5 nm, which is shallower than the corresponding depth in the non-contact area. Finally, a region with reduced water retention is observed, characterized by molecular ion fragments corresponding to the coating (92MoS2— to 92MoS3—).
3.3. Implications for tribology
The regions with distinct water mobility discussed above for the non-contact area and the wear track can directly impact the friction performance of MoS2 as a solid lubricant. To connect the findings of this work with published studies, two scenarios explored in the tribology community for ultra-thin MoS2 coatings, where top surface phenomena are relevant, are discussed in the following: the first one is related to nanotribology and the second one is related macroscale tribology.
In nanotribology, MoS2 finds its application in micro- and nano-electro-mechanical systems (MEMS/NEMS), where fine control of friction is critical. Studies on ultra-thin MoS2 films deposited by atomic layer deposition (ALD), ranging from 1 to 10 layers, with an interplanar spacing of ~0.65 nm for (002) basal planes, have been conducted using atomic force microscopy (AFM) operated in contact mode. The normal loads applied to the tip and the scan velocities ranged from 5-50 nN (∼5-7 GPa maximum Hertzian pressure) and 0.3-10 µm/s[22–24], respectively. It was observed that the substrate effect on friction behavior gradually disappeared after 6 layers (~3.9 nm) when measured in air conditions, particularly under a humid environment (relative humidity was kept at 40-50%)[22].
In macroscale tribology, MoS2 is used in mechanical applications such as bearing, gears and machining tools, where wear rate and friction response are of critical consideration due to usually high life cycle requirements. A study on ultra-thin MoS2 films deposited by combined ALD and H2S annealing has been conducted using a flexure-based bidirectional reciprocating tribometer[25]. The normal load applied to the 3.2 mm diameter 440C steel ball and the sliding velocity were 100 mN (∼407 MPa maximum Hertzian pressure) and 1 mm/s, respectively. It was found that converted-ALD MoS2 coatings of thickness ~5-10 nm (~8-16 layers) had an estimated wear life of < 1 × 10−6 mm3/N m when tested in a dry N2 atmosphere (O2 < 50 ppm, H2O < 50 ppm). This translates to a single MoS2 layer being removed after approximately 16 sliding passes.
These examples illustrate how frictional phenomena in the first few nanometers of MoS2 coatings are relevant to tribology, as depicted previously in Figures 3 and 6, in which the characteristics of water transport in the first 6.5 nm are shown. In light of this, the findings of this work offer further guidelines for improving the tribological performance of MoS2 even after aging in humid conditions by tuning the characteristics of the first 6.5 nm. Here, we report two potential scenarios:
• In cases where the MoS2 coating is deposited and stored in humid conditions, pre-annealing in vacuum after deposition decreases the overall water uptake (including with postannealing), which proves beneficial for friction performance.
• In situations where the MoS2 coating is deposited, tribologically stressed, and then stored in humid conditions, preannealing in vacuum after tribological testing increases the overall water uptake (including with post-annealing), thereby detrimentally affecting friction performance.
Once the top 6.5 nm have undergone wear, the tribological performance is contingent upon deeper layers. The overarching observation in both non-contact areas and wear tracks, irrespective of post-annealing treatment, is that pre-annealing in vacuum leads to an increased water uptake after 55 days, thus proving detrimental to friction performance. While pre-annealing may potentially remove latent water and be beneficial for friction performance in the first few nanometers, its long-term consequence is the increase of water content inside the MoS2 coating, which, in turn, worsens the friction behavior following wear. Consequently, it is advised to not pre-anneal the MoS2 coating if an extended long life cycle is anticipated under field conditions.
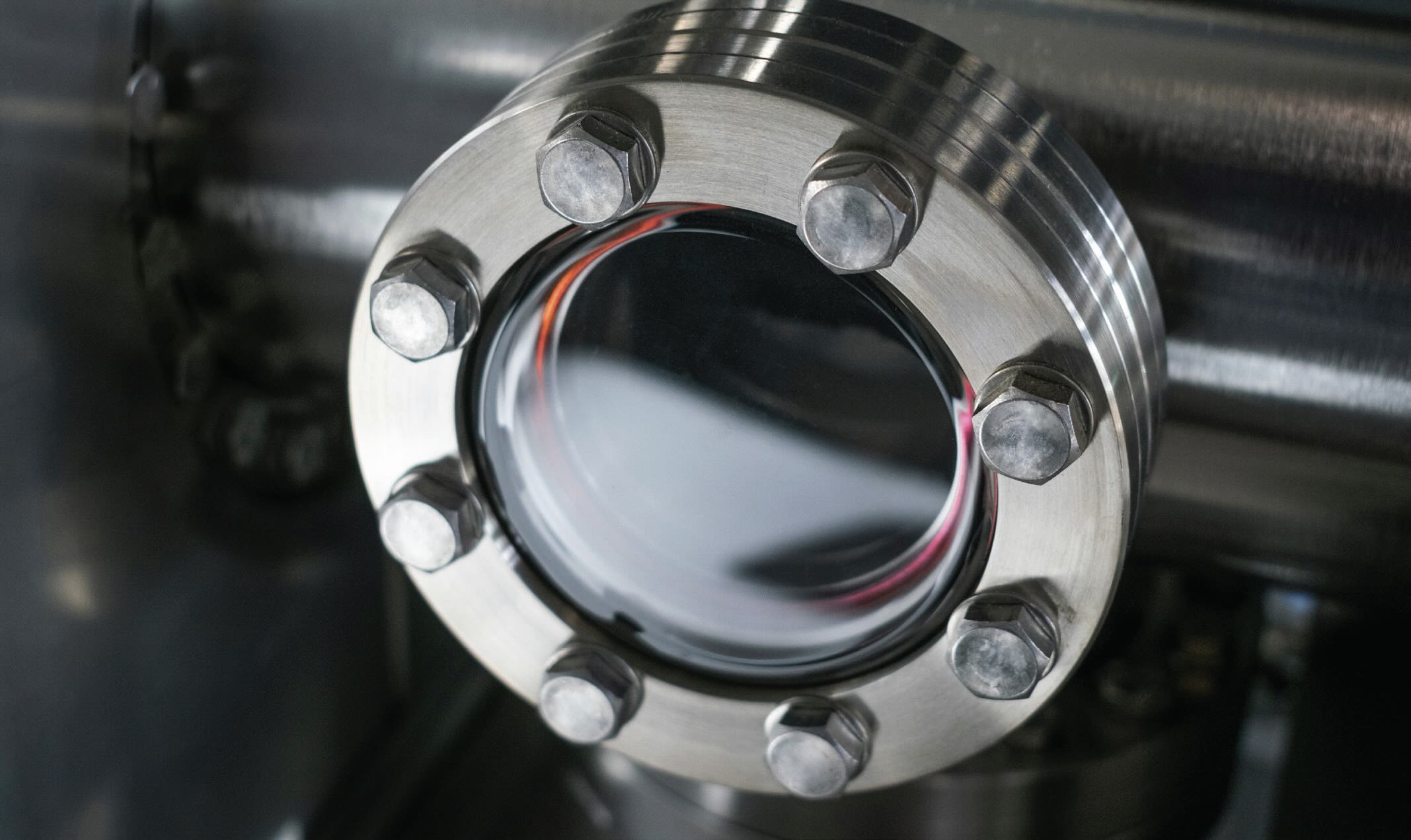
4. Conclusions
This study sheds light on the depth distributions of molecular ion fragments corresponding to water (OH—) and MoS2 (92MoOx— and 92MoSx—) within both the non-contact area and wear track of sputtered- MoS2 coatings. Notable differences were observed for coatings aged in humid air with pre- and post-annealing treatment, with the pre-annealing treatment leading to generally increased water diffusion into the bulk while reducing near-surface water content compared to untreated (as-received) specimens. The depth distributions of 92MoOx– and 92MoSx– revealed a gradient of chemistry within the coating. This change in chemical environment with depth, coupled with changes in microstructure upon annealing, significantly impacted water diffusion and retention behavior within the coating. The findings offer valuable insights into how frictional phenomena in the first few nanometers of MoS2 coatings are crucial for understanding and optimizing tribological performance. Recommendations for optimizing friction performance in humid conditions were provided, emphasizing the need to consider the initial layers’ characteristics and the long-term consequences of pre-annealing treatments. Further studies are needed to establish quantitative links between the microstructure of as-deposited MoS2, water diffusivity in the near-surface region before and after tribological testing, and subsequent tribological performance under varying environmental conditions.
Acknowledgments
The authors would like to thank STLE for the support provided by the 2023 Elmer E. Klaus Fellowship. This work was also funded by the Laboratory Directed Research and Development program at Sandia National Lab., a multi-mission laboratory managed and operated by National Technology and Engineering Solutions of Sandia, LLC., a wholly owned subsidiary of Honeywell International, Inc., for the US DOE’s National Nuclear Security Administration under contract DENA0003525. The authors also convey special thanks to people who helped conduct and discuss the results: Robert Chrostowski from the Texas Materials Institute – The University of Texas at Austin (Austin, Texas); Tomas F. Babuska, John F. Curry, and Michael T. Dugger from the Material, Physical and Chemical Sciences Center – Sandia National Laboratories (Albuquerque, N.M.).
1. Donnet, C., Martin, J.M., Le Mogne, T., Belin, M.: The origin of super-low friction coefficient of MoS2 coatings in various environments. Tribol. Ser. 27, 277–284 (1994). https://doi.org/10.1016/S0167-8922(08)70317-1
2. Hilton, M.R., Fleischauer, P.D.: Applications of solid lubricant films in spacecraft. Surf. Coatings Technol. 54–55, 435–441 (1992). https://doi.org/10.1016/S0257-8972(07)80062-4
3. Roberts, E.W.: Ultralow friction films of MoS2 for space applications. Thin Solid Films. 181, 461–473 (1989). https://doi.org/10.1016/0040-6090(89)90515-4
4. Vazirisereshk, M.R., Martini, A., Strubbe, D.A., Baykara, M.Z.: Solid lubrication with MoS2: A review. Lubricants. 7, (2019). https://doi.org/10.3390/LUBRICANTS7070057
5. He, X., Liu, Z., Ripley, L.B., Swensen, V.L., Griffin-Wiesner, I.J., Gulner, B.R., McAndrews, G.R., Wieser, R.J., Borovsky, B.P., Wang, Q.J., Kim, S.H.: Empirical relationship between interfacial shear stress and contact pressure in micro- and macro-scale friction. Tribol. Int. 155, 106780 (2021). https://doi.org/10.1016/j.triboint.2020.106780
6. Sun, Y., Wang, Y., Wang, E., Wang, B., Zhao, H., Zeng, Y., Zhang, Q., Wu, Y., Gu, L., Li, X., Liu, K.: Determining the interlayer shearing in twisted bilayer MoS2 by nanoindentation. Nat. Commun. 13, 1–9 (2022). https://doi.org/10.1038/s41467-022-31685-7
7. Khare, H.S., Burris, D.L.: The effects of environmental water and oxygen on the temperature-dependent friction of sputtered molybdenum disulfide. Tribol. Lett. 52, 485–493 (2013). https://doi.org/10.1007/s11249-013-0233-8
8. Babuska, T.F., Krick, B.A., Argibay, N., Dugger, M.T., Chandross, M., Curry, J.F.: Revisiting the dwell effect on friction behavior of molybdenum disulfide. Wear. 526–527, 204876 (2023). https://doi.org/10.1016/j.wear.2023.204876
9. Mao, Q., Zhang, Y., Kowalik, M., Nayir, N., Chandross, M., van Duin, A.C.T.: Oxidation and hydrogenation of monolayer MoS2 with compositing agent under environmental exposure: The ReaxFF Mo/Ti/Au/O/S/H force field development and applications. Front. Nanotechnol. 4, 1–18 (2022). https://doi.org/10.3389/fnano.2022.1034795
10. Ghuman, K.K., Yadav, S., Singh, C.V.: Adsorption and dissociation of H2O on monolayered MoS2 edges: Energetics and mechanism from ab initio simulations. J. Phys. Chem. C. 119, 6518–6529 (2015). https://doi.org/10.1021/jp510899m
11. Ataca, C., Ciraci, S.: Dissociation of H2O at the vacancies of single-layer MoS2. Phys. Rev. B -Condens. Matter Mater. Phys. 85, 1–6 (2012). https://doi.org/10.1103/PhysRevB.85.195410
12. Levita, G., Righi, M.C.: Effects of Water Intercalation and Tribochemistry on MoS2 Lubricity: An Ab Initio Molecular Dynamics Investigation. ChemPhysChem. 18, 1475–1480 (2017). https://doi.org/10.1002/cphc.201601143
13. Colas, G., Saulot, A., Philippon, D., Berthier, Y., Leonard, D.: Time-of-Flight Secondary Ion Mass Spectroscopy investigation of the chemical rearrangement undergone by MoS2 under tribological conditions. Thin Solid Films. 588, 67–77 (2015). https://doi.org/10.1016/j.tsf.2015.04.051
14. Chrostowski, R., Curry, J.F., Dugger, M.T., Molina, N., Babuska, T.F., Celio, H., Dolocan, A., Mangolini, F.: Spectroscopic Evaluation of Surface Chemical Processes Occurring in MoS2 upon Aging. ACS Appl. Mater. Interfaces. 15, (2023). https://doi.org/10.1021/acsami.3c06737
15. Zhu, Z., Shutthanandan, V., Engelhard, M.: An investigation of hydrogen depth profiling using tof-SIMS. Surf. Interface Anal. 44, 232–237 (2012). https://doi.org/10.1002/sia.3826
16. Colas, G., Saulot, A., Philippon, D., Berthier, Y., Léonard, D.: Tribochemical Competition within a MoS2/Ti Dry Lubricated Macroscale Contact in Ultrahigh Vacuum: A Time-of-Flight Secondary Ion Mass Spectrometry Investigation. ACS Appl. Mater. Interfaces. 10, 20106–20119 (2018). https://doi.org/10.1021/acsami.8b02999
17. Tyler, B.J., Rayal, G., Castner, D.G.: Multivariate analysis strategies for processing ToF-SIMS images of biomaterials. Biomaterials. 28, 2412–2423 (2007). https://doi.org/10.1016/j.biomaterials.2007.02.002
18. Ziegler, J.F., Ziegler, M.D., Biersack, J.P.: SRIM -The stopping and range of ions in matter (2010). Nucl. Instruments Methods Phys. Res. Sect. B Beam Interact. with Mater. Atoms. 268, 1818–1823 (2010). https://doi.org/10.1016/j.nimb.2010.02.091
19. Pardee, R.P.: The effect of humidity on low-load frictional properties of a bonded solid film lubricant. ASLE Trans. 15, 130–142 (1972). https://doi.org/10.1080/05698197208981409
20. Bobbitt, N.S., Chandross, M.: Interactions of Water with Pristine and Defective MoS2. Langmuir. 38, 10419–10429 (2022). https://doi.org/10.1021/acs.langmuir.2c01057
21. Wang, R., Wang, X., Zuo, Z., Ni, S., Dai, J., Wang, D.: Adsorption Equilibrium and Mechanism and of Water Molecule on the Surfaces of Molybdenite (MoS2) Based on Kinetic Monte-Carlo Method. Molecules. 27, (2022). https://doi.org/10.3390/molecules27248710
22. Yang, J., Liu, L.: Nanotribological properties of 2-D MoS2 on different substrates made by atomic layer deposition (ALD). Appl. Surf. Sci. 502, 1–7 (2020). https://doi.org/10.1016/j.apsusc.2019.144402
23. Yang, J., Xu, X., Liu, L.: Plasma-assisted friction control of 2D MoS2 made by atomic layer deposition. Nanotechnology. 31, (2020). https://doi.org/10.1088/1361-6528/ab978c
24. Huang, Y., Liu, L., Lv, J., Yang, J., Sha, J., Chen, Y.: MoS2 solid-lubricating film fabricated by atomic layer deposition on Si substrate. AIP Adv. 8, (2018). https://doi.org/10.1063/1.5021051
25. Babuska, T.F., Dugger, M.T., Walczak, K.A., Lu, P., Schwartzberg, A., Aloni, S., Kuykendall, T.R., Curry, J.F.: Tribological behavior of few-nanometer-thick MoS2 prepared by low-temperature conversion of atomic layer deposited MoOx films. Surf. Coatings Technol. 471, 129884 (2023). https://doi.org/10.1016/j.surfcoat.2023.129884