Meet the Presenter
Dive into the world of tribocorrosion with this article, based on the webinar titled Tribocorrosion Aspects of Biomedical Implants: Where are we now? Hosted by the American Society of Mechanical Engineers’ (ASME) Tribology Division and presented by Dr. Mathew Mathew on Feb. 22, 2023, the session explored basic tribocorrosion aspects of implants and the effect of infectious and inflammatory conditions on the cellular viability of osteoblast cells and corrosion aspects of the implants under macrophages challenged by these conditions. Courtesy of STLE, this article captures the core insights from this ASME-organized event. For more technical content from the ASME Tribology Division Webinar Series, visit the ASME Tribology Division’s website at www.asme.org/get-involved/groups-sections-and-technical-divisions/technical-divisions/technical-divisions-community-pages/tribology-division.
Dr. Mathew Mathew is the Cedric W. Blazer endowed professor of biomedical science in the Department of Biomedical Science at the University of Illinois College of Medicine (UICOM), Rockford, Ill., where he leads the Regenerative Medicine and Disability Research Lab. His major research focus is in the area of simulation of human artificial joints and the biomechanics and tribocorrosion of implanted materials used in dentistry and orthopedics. He is an editor in chief of the journal Bio- and Tribo- Corrosion (https://link.springer.com/journal/40735), and he also is a co-founder of the Institute of Biomaterials, Tribocorrosion, Nano, and Regenerative Medicine (IBTN, https://ibtn.lab.uic.edu/). You can reach him at mtmathew@uic.edu.
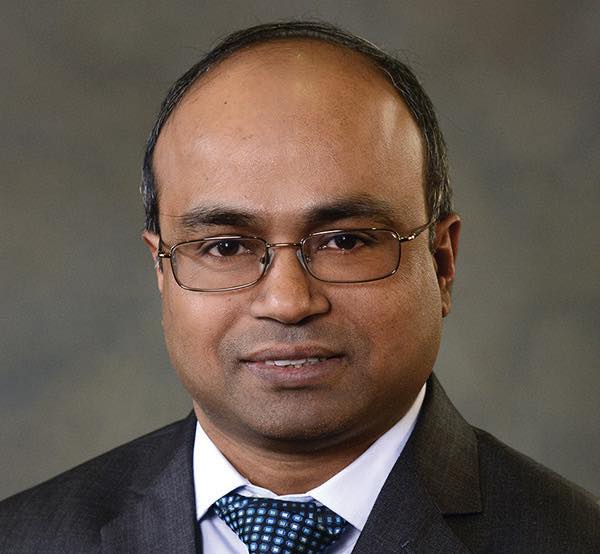
Dr. Mathew Mathew
KEY CONCEPTS
•
Implants function mechanically like other mechanical joints, but within a biochemical environment.
•
Wear particles, ions, cells and fluids can interact with implants and tissues to set up a cycle of corrosion and wears.
•
Tribocorrosion modeling and prediction remain active areas of research.
From a mechanical engineering standpoint, biomedical implant joints are like other mechanical joints. Shoulder and hip joints are like a ball and socket, for example
(see Figure 1), and the elbow is like a hinge joint. These joints must function properly for a person to stand, walk and conduct daily activities, but aging, disease or injury can interfere with joint functioning. Common causes of joint pain include reactive arthritis, septic arthritis, rheumatic fever, infections, malignancies like acute lymphoblastic leukemia, mechanical causes like slippage of the upper-femoral epiphysis (the widened end of the bone), avascular necrosis or ordinary wear and tear. Trauma, like slipping and falling on an icy sidewalk or sustaining a sports injury, can cause painful joint fractures.
Figure 1. Hip implant components include the stem, head and cup with polymer liner. Figure courtesy of Dr. Jean Geringer, Ecole des Mines de Saint-Etienne.
This article is based on a webinar presented by Dr. Mathew Mathew, Cedric W. Blazer endowed professor of biomedical science in the Department of Biomedical Science at the University of Illinois College of Medicine (UICOM), Rockford, Ill., and the Tribology Division of the American Society of Mechanical Engineers (ASME) titled Tribocorrosion Aspects of Biomedical Implants: Where Are We Now? See Meet the Presenter for more information.
In a recent survey, almost half of adult respondents over the age of 65 reported doctor-diagnosed arthritis.
1 One 2006 study predicted that by the year 2030, about 67 million Americans ages 18 years or older will be diagnosed with some form of arthritis.
2,3
There are many ways we can treat diseased joints, from the temporomandibular joint in the jaw to knee and hip replacements. The first line of treatment usually involves physical therapy and medications, but if these are not effective, repair surgery may be necessary. If none of these treatments works, the joint must be replaced. Biomedical implants are generally a good solution to joint or dental problems that cannot be treated by other means, but bone implant failure rates can exceed 10%.
4
How do these implants fail? Infection and inflammatory reactions are the main cause of implant failure, but there are many other reasons, including the design of the prosthesis and the patient’s height and weight, joint loading and activities
(see Figure 2). To reduce the chance of failure, the surgeon must properly align the joint and the soft-tissue balance, ensuring that the joint remains aligned during extension and flexion. Failure rates also depend on the prosthetic material. Replacement joint components generally involve one or more types of metal, including aluminum, magnesium, titanium or zirconium. They also can contain alloys, including CoCrMo (68% cobalt, 25% chromium, 7% molybdenum) or Ti6Al4V (90% titanium, 6% aluminum, 4% vanadium), as well as other materials including silicon, ceramics and polymers.
Figure 2. A variety of factors can cause hip implants to fail.
Mathew and his collaborators collect failed implants that have been removed from patients and study the causes of their failure. The main issues with commonly used metal implants include inflammation, osteolysis (bone degeneration), crack initiation, early fracture and the release of metal ions and wear debris. At present, the average lifespan of a hip joint implant is 12 to 15 years, although these implants can last longer or fail earlier.
Implants can fail because of a buildup of corrosion and wear products, which can damage the surrounding tissue. On a microscale, stem tapers can experience intergranular corrosion, with the formation of hard phases along grain boundaries. An acid environment in the mouth (e.g., from drinking carbonated beverages), combined with masticating forces and microbial products, can affect the lifetime of dental implants and the health of the surrounding tissue
(see Figure 3). Dental implants can also be corroded by certain medications or metal-to-metal contact (galvanic corrosion).
Figure 3. Acids, microbes and galvanic corrosion can damage the metal components of dental implants. Figure courtesy of Dr. Cortino Sukotjo, University of Illinois Chicago (UIC) Dental School.
The role of tribocorrosion in implant failure
Unlike mechanical joints in an industrial setting, biomedical joints face the biological aspects of their environment, in addition to issues arising from the joint material and applied stresses. Thus, for a given implant material, the body’s reaction to the foreign material of the implant, local tissue reactions, osteolysis and infection, along with mechanical wear caused by physical activity, can accelerate implant corrosion.
In early orthopedic literature, Dr. Jeremy Gilbert and others called these effects “mechanically assisted corrosion.”
5 In fact, the classical definition of tribocorrosion is “an irreversible transformation of a material resulting from simultaneous physicochemical and mechanical surface interactions in a tribological contact.”
6 Tribocorrosion comes about as a result of friction and wear. This type of corrosion can generate particles and ions that accelerate the degradation of an implant. Biomedical implants introduce several kinds of interfaces where tribology is important. For example, in a hip joint, a smooth nonstick surface is desirable for the interface between head of the implant and the hip socket, but there must be a strong adhesive bond between the stem and the surrounding bone. After about two years of wear, there is always a fretting motion at the stem–bone junction. Fretting also occurs at the modular junction between the joint head and neck. These mechanical and tribological aspects are similar to joints in a mechanical setting. However, in a biological setting, the implant is surrounded by cells like macrophages, osteoblasts, monocytes and fibroblasts, which also interact with the implant
(see Figure 4).
Figure 4. Total joint replacements (TJRs) experience mechanical wear, as well as interacting with cells and biological fluids. Figure courtesy of Dr. Mathew Mathew.
Tribocorrosion research: Basics and progress
Any examination of tribocorrosion must take force and movement into account, along with lubrication and electrochemical potentials. The mechanical data include friction, surface damage and fretting loops. Electrochemical data include evolution of current and potential as a function of time. Tribological studies require moving parts, but typical corrosion studies don’t include motion, so the integration of these two disciplines will be a complex process.
The progress in tribocorrosion is closely associated with contributions of the work of the pioneers in this field, namely, Prof. JP. Celis (Belgium), Prof. MM. Stack (UK), Prof. Stefano Mischler (Switzerland), Prof. Robert Wood (UK), Prof. Anne Neville (UK), Prof. Jean Geringer (France), Dr. Yu Yan (China), Dr. Manish Roy (India) and Prof. Luis Rocha (Portugal) and many others. Prof. MM. Stack is leading a network of tribocorrosion researchers from all over the world (
https://www.tribos-strath.com/) and conducting an annual tribocorrosion symposium. The workshop and symposium at the EU corrosion conference (EUROCORR) led by Prof. Mischler and team has been beneficial to many new researchers and scientists in tribocorrosion.
The literature records many researchers’ attempts to develop a protocol or model for the synergistic analysis of wear and corrosion. For example, a collaborative work with Dr. Orlando Auciello at The University of Texas at Dallas demonstrated that ultrananocrystalline diamond (UNCD) coatings behave differently when friction is involved. In the absence of friction, the corrosion potential of UNCD as a function of time is similar to that for noble metals like gold or silver. That is, the UNCD coatings show little susceptibility to moisture-induced oxidation or corrosion
(see Figure 5). Once friction enters the picture, however, there is a drop in potential— corrosion can begin at a lower electrochemical potential. As long as the friction levels are low, the coating holds up very well. But after several seconds under friction, the coating fails, the friction increases and the corrosion potential further decreases with the rubbing on the substrate material. The material corrodes and forms protective passivating deposits, which then wear away again, allowing corrosion to resume. Thus, the corrosion and tribology processes go hand in hand.
Figure 5. Ultrananocrystalline diamond (UNCD) coatings in artificial saliva (pH 6.5) show little susceptibility to corrosion until several seconds after friction begins. Corrosion forms passivating deposits, which wear away and corrosion begins again. Figure courtesy of Dr. Orlando Auciello, The University of Texas at Dallas.
Tribology-only studies don’t provide information about corrosion, and corrosion-only studies don’t provide information about the mechanical aspects of failure, so both studies must be combined. Data that combine both aspects can show where corrosion is increasing in a biological environment. The effects of passive films in the body like solution-resistant films or biofilms, and the effects of proteins and bone cells, can be studied in a controlled environment in the lab. These studies show where metal ions are released and metal debris is generated, as well as the underlying mechanisms of implant failure.
Hip simulator
A pin-on-ball tribometer that Mathew modified to function as a hip simulator tests friction for a hip head made of cobalt chromium, and it simulates a walking cycle in a bovine calf serum that mimics synovial fluid
(see Figure 6). Electrodes monitor corrosion during the simulations. These simulations show that an increase in current increases friction, demonstrating the interaction of mechanical and electrochemical effects. To get meaningful data from these experiments, the challenge is to understand the mechanical synergism and the individual contributions from wear and corrosion.
Figure 6. A tribometer equipped with electrodes measures tribocorrosion effects during a simulated walking cycle for an artificial hip head immersed in fluid. Figure courtesy of Dr. Markus Wimmer, Rush Arthritis and Orthopedics Institute.
Mathew also has looked at surfaces after tribocorrosion and used inductively coupled plasma–mass spectrometry (ICP-MS) analysis to identify the particles released for a given number of cycles. He found CoCrMo particles/ions released during testing. He measured the total weight loss using profilometry and ICPMS data. He notes that, when studying biomedical devices, it’s important to bear in mind that mechanical effects and corrosion affect each other synergistically. Another important factor is understanding the types of corrosion mechanisms in effect
(see Figure 7). Tribological effects like abrasion, fretting, fatigue and strain happen alongside electrochemical corrosion effects like dissolution, passivation and de-passivation and pitting.
Figure 7. Tribological and corrosion mechanisms work together to alter a material’s microstructure. Figure courtesy of professor Margaret Stack, University of Strathclyde, UK.
Current research and future directions
A wide variety of tribocorrosion models is available to study to estimate the synergistic interaction of wear and corrosion.
7,8,9 However, better simulations of tribocorrosion processes in implants are needed. The current state of the art has several limitations, including an understanding of how corrosion accelerates wear under biological condition as well as the role of material microstructure on tribocorrosion processes. Tribocorrosion and biological systems must be interfaced to study the toxicity of tribocorrosion products. We can then put this knowledge to use in predicting and minimizing tribocorrosion processes in implants.
Mathew and his colleagues are actively researching ways to minimize this type of corrosion process and to predict tribocorrosion processes in implants. In their lab, they simulate human activities like walking, jogging and running to observe fretting behavior. They have observed that friction energy increases as a function of displacement, and they observed the evolution of an electrochemical potential. The greater the displacement, the steeper the corrosion potential drop, and the more easily corrosion occurs.
Recently, they repeated these experiments on a more realistic scale, with a smaller magnitude of motion (5 micrometers or less), using a new fretting-corrosion simulator that they developed
(see Figure 8). This is an NIH R01 collaborative work between University of Illinois Chicago (UIC) and the Rush Arthritis and Orthopedics Institute, Dr. Hannah Lundberg and Dr. Robin Pourzal, STLE member Dr. Markus Wimmer, Dr. Alfons Fisher and Dr. Joshua Jacobs. Because of the small scale, they couldn’t observe the effects directly, but the data showed them the interactions between the friction energy and the material loss.
Figure 8. This simulator measures fretting-corrosion at a scale of 5 micrometers or less. Figure courtesy of Dr. Kai-yuan Cheng, University of Illinois College of Medicine at Rockford, and Yani Sun, UIC.
Mathew’s group also is studying metal hip taper junction joints; running tests that simulate the motions of a person getting up, standing and walking for a new implant; an implant after two years; an infected implant; and an implant after 10 to 15 years. They recently observed that cell secretions can accelerate corrosion during a collaborative study on how and where cells migrate to this taper junction. This collaboration involved Dr. Xue-Jun Li (UICOM-Rockford), Dr. Mike McNallan (UIC-Chicago) and Dr. Mark Barba (OrthoIllinois, Rockford), and several former postdoc researchers from Mathew’s lab: Dr. Divya Bijukumar, Dr. Ravi Badhe, Dr. Hemalatha Kanniyappan, Dr. Kai Cheng and Dr. Govindaraj Perumal and other graduate students from the UIC Master of Science in Medical Biotechnology Program. Using simulations of macrophages, they found that when wear particles or ions challenge the macrophages, these immune cells change their morphology and functions, and they observed how this affects implant failure
(see Figure 9).
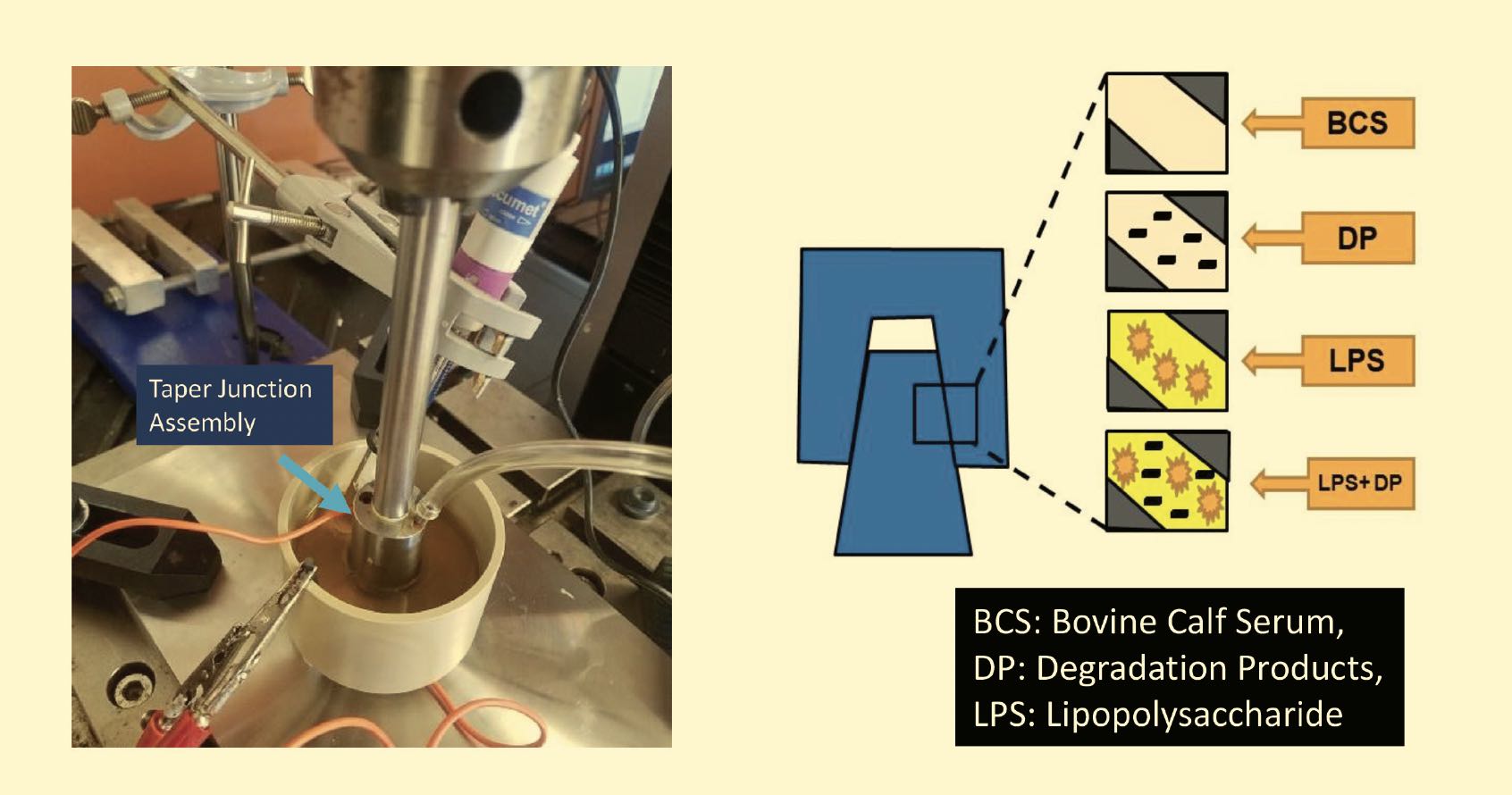
Figure 9. Hip taper junction tests simulate standing up, standing and walking for new and aged implants and infected implants. Figure courtesy of Dr. Kai-yuan Cheng, University of Illinois College of Medicine at Rockford, and Jacob Manthe, UIC.
Mathew’s former doctorate student, Dr. Mostafa Ahamad, is studying titanium dental implants, specifically the effects of titanium ions and particles. Infectious conditions and metal ions can trigger a release of inflammatory peroxides and lactic acid into the interface between the implant and the gingival tissue, triggering an immune response that increases the corrosion of the titanium implants. Synergistic effects can lead to cycles of accelerating corrosion and infection that eventually result in implant failure. Dr. Cortino Sukotjo, Dr. Chen and Dr. George (UIC College of Dentistry) and Dr. Valentim Barao (IBTN) assisted with this collaborative project.
Mathew, Dr. Pourzal and colleagues also are studying the effect of CoCrMo microstructure on cell-accelerated corrosion using tribocorrosion tests in simulated joint fluid. When they compared banded and nonbanded microstructures, they observed that the banded structure that had been challenged with particles or ions experienced a higher material loss than the nonbanded structure, and the challenger particles or ions had a higher total charge compared to the control. This results in more damage to the banded structure, so microstructure plays a role in this fretting damage.
They have extended this work to a study of toxicity effects; they pump solution into a microfluidic channel where cells are exposed to metal particles. Their dynamic hip microfluidic bioreactor (DHMB) test system, which integrates a hip simulator with a bioreactor and microfluidic system, simulates a person walking. They use a microscope mounted with the microfluidic system to watch the interactions between particles and cells and observe the tribocorrosion effects.
Another of their studies involves using several types of cells or simulations to study toxicity effects. One such study focuses on neurotoxicity, to address recent reports of implant patients experiencing vision impairment and numbness. In a labscale study, they cultured neuron cells and allowed them to move through a microfluidic channel to observe cell viability as a function of speed. Not surprisingly, toxicity increases when the particles spend more time in the microfluidic system. This work shows how
in vivo degradation takes place in a dynamic system.
Mathew’s group is collaborating with several investigators, including STLE member Dr. Min Zou at the University of Arkansas, to develop coatings that minimize the tribocorrosion process. These coatings must have desirable mechanical properties (e.g., resisting delamination at the interface) as well as having minimal toxic effects. In collaborating with Dr. Oral Ebru (Harvard Medical School), they are looking at adding an antioxidant like vitamin E, carvacrol or eugenol to the coating to reduce corrosion and potential risk of implant generated toxicity.
Prediction studies
Mathew’s research also is focusing on predicting implant failures, with the aim of catching failures in the early stages by predicting the most likely type of corrosion. Mathew’s group, in collaboration with Dr. Shalini Prasad and Danieli Rodrigues (UT Dallas), developed small microchip sensors that they use to detect injected particles in the bloodstream of an animal model. For comparison, they collect a blood sample and compare the sensor readings with the laboratory analysis of the particles from the blood sample. In a human implant patient, this type of sensor could signal if cobalt or chromium levels were increasing in the patient’s bloodstream, alerting their physician to take corrective action before the problem becomes more serious. Such early warnings could extend the life of the implant as well as helping to prevent negative effects on the patient’s health.
Another technique, acoustic emission (AE), is showing promise as a very noninvasive technique for the early detection of failure modes in total hip replacements. Mathew’s group is collaborating with other researchers, Dr. Didem Ozevin and Dr. Kharma Foucher (UIC) and Dr. Srinivas Pai (NMAM Institute of Technology, India) to develop a sensing system based on an AE model, which detects abnormal vibrations that can signal increases in wear. Postdoctoral researcher Dr. Remya Ampadi and colleagues are collecting tribocorrosion data to compile a database for machine learning, which they hope to use for an artificial intelligence system that detects risks early on.
10 Their goal is to develop a continuous monitoring system where the patient can go about their daily activities and then send AE sensor data from their implant to their medical practitioner using a cell phone or other device.
Although most biomedical implants perform well, concern over the failures that do occur have prompted Mathew’s studies on the role of tribocorrosion, where wear and corrosion interact synergistically. Linking tribocorrosion and the biological system is essential to understanding corrosion of implanted devices. Much research is still required to enable the prediction and minimization of tribocorrosion, with the goal of extending the lifetime of biomedical implants and guaranteeing their performance.
REFERENCES
1.
Fallon, E. A., et al. (Oct. 13, 2023), “Prevalence of diagnosed arthritis — United States, 2019–2021,” U.S. Centers for Disease Control, Morbidity and Mortality Weekly Report, doi: 10.15585/mmwr.mm7241a1.
2.
Hootman, J. M. and Helmick, C. G. (2006), “Projections of US prevalence of arthritis and associated activity limitations,”
Arthritis & Rheumatism, 6 (54), 1, pp. 226-229, doi: 10.1002/art.21562
3.
U.S. Centers for Disease Control, “Arthritis related statistics.” Available
here.
4.
Peres, I., et al. (2022), “Multifunctional smart bone implants: Fiction or future?—A new perspective,”
Front. Bioeng. Biotechnol., 10, 921081, doi: 10.3389/fbioe.2022.912081.
5.
Gilbert, J. L. (2006), “Mechanically assisted corrosion of metallic biomaterials,”
ASM Handbook, Corrosion: Environments and Industries, ASM International, 13C, doi:
https://doi.org/10.31399/asm.hb.v13c.9781627081849, ISBN electronic: 978-1-62708-184-9.
6.
Ladolt, D., Mischler, S. and Stemp, M. (2001), “Electrochemical methods in tribocorrosion: a critical appraisal,”
Electrochim. Acta, 46 (24-25), pp. 3913-3929, doi: 10.1016/S0013-4686(01)00679-X.
7.
Stack, M. M. and Mathew, M. (2003), “Micro-abrasion transitions of metallic materials,”
Wear, 255 (1), pp. 14-22, doi: 10.1016/S0043-1648(03)00204-7.
8.
Mischler, S. (2013), “Sliding tribo-corrosion of passive metals: Mechanisms and modeling,” In: Blau, P.J., Celis J.P., Drees D., editors,
Tribo-Corrosion: Research, Testing, and Applications, Atlanta, Ga., ASTM International; pp. 1-18.
9.
Mellado-Valero, A., Muñoz, A. I., Pina, V. G. and Sola-Ruiz, M. F. (2018), “Electrochemical behaviour and galvanic effects of titanium implants coupled to metallic suprastructures in artificial saliva,”
Materials, 11 (2), 171, doi:10.3390/ma11010171.
10.
Lee, C., et al. (2020), “Non-invasive early detection of failure modes in total hip replacements via acoustic emission techniques,” Poster 1121, Orthopaedic Research Society, 2020 Annual Meeting, click
here.
Nancy McGuire is a freelance writer based in Albuquerque, N.M. You can contact her at nmcguire@wordchemist.com.