Translational cartilage tribology: How close are we to physiologically relevant benchtop articular cartilage testing?
Margot S. Farnham and Christopher Price | TLT Fellowship Research February 2020
Department of Biomedical Engineering, University of Delaware, Newark, DE 19716
Online only in TLT Archives
Klaus Fellowship paper focuses on bridging the tribometry gap
The Elmer E. Klaus Fellowship is awarded annually to graduate and undergraduate students, respectively, who have an interest in pursuing a career in tribology. As a requirement for receiving an STLE scholarship, students participate in a tribology research project and submit a report summarizing their research. For more information about the Klaus Fellowship, visit www.stle.org.
The 2019 recipient of The Elmer E. Klaus Fellowship Award is Margot Farnham, a fourth-year biomedical engineering student working the Price Cartilage Mechanobiology Lab at the University of Delaware. Her research focuses on understanding articular cartilage’s tribological and mechanobiological properties, with a special interest on mechanically damaged and osteoarthritic cartilage. You can reach her at mfarnham@udel.edu.
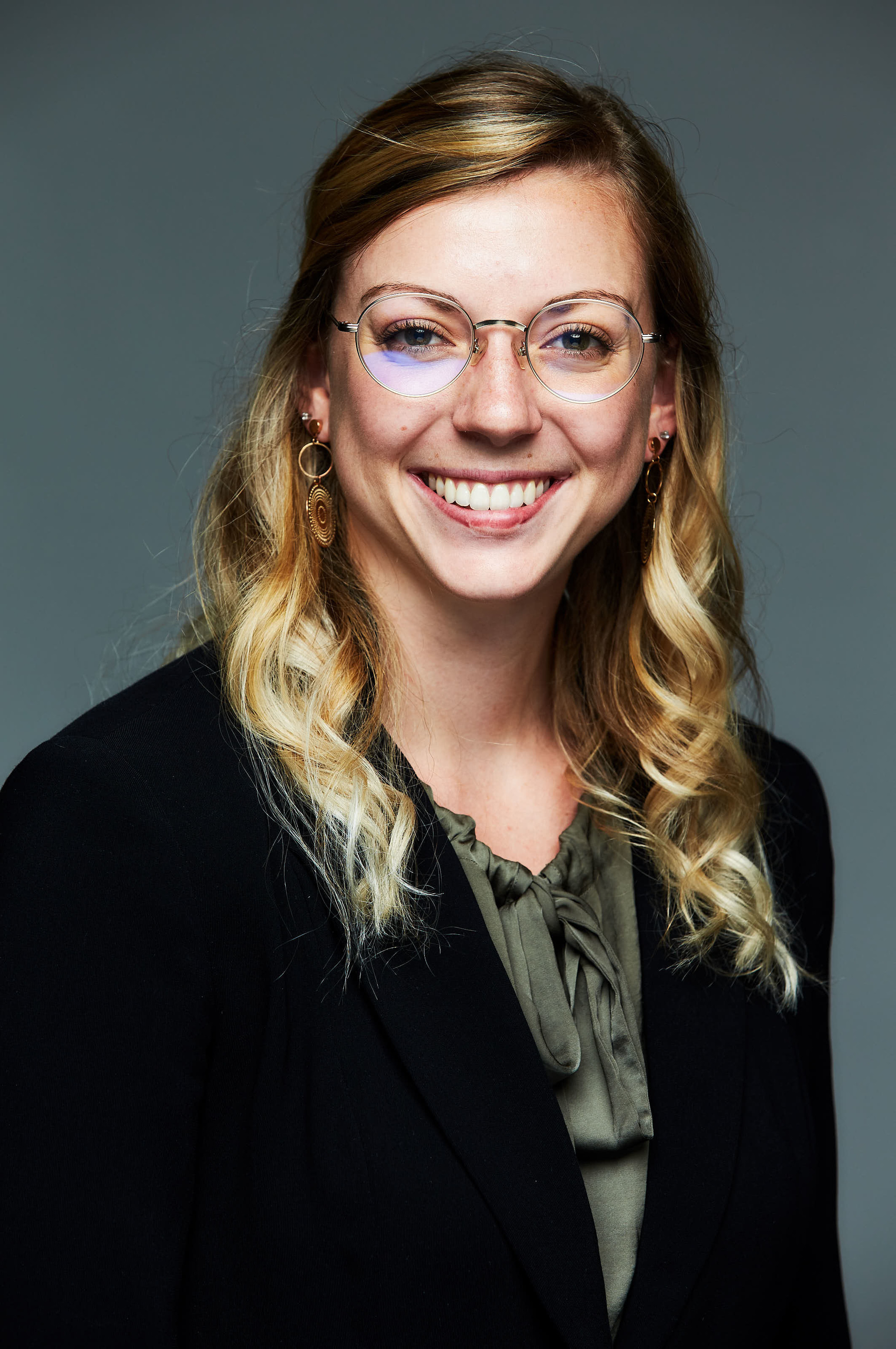
Margot Farnham
I. Benchtop lubrication testing: have we oversimplified cartilage’s complex tribological behavior?
Articular cartilage is an extremely resilient yet complex material; it promotes remarkably low friction motion throughout the millions of articulations our joints endure annually. Cartilage can be described as a bi- or multi-phasic tissue
3, with ~80% of its volume consisting of water held in place by a solid collagen and proteoglycan matrix. This phenomenal material has been of interest to researchers and engineers for nearly a century
1,2, and to both investigate cartilage’s lubrication properties and to develop theories explaining cartilage lubrication, mechanists have traditionally looked to two common testing configurations: those containing stationary contact areas (SCA)
4–8 and those containing migrating contact areas (MCA)
9,10 (Figure 1).
The MCA configuration (Figure 1, top center) consists of a semi-spherical probe brought into contact with a relatively flat cartilage surface
10,11. This ‘probe’ is used to apply compressive stresses in combination with sliding to the surface of the tissue, allowing for friction measurements. The MCA configuration helped confirm the
interstitial lubrication8,10,12–15 theory of cartilage, originally proposed by Mow and colleagues in the 1980’s (Figure 1, bottom center). Interstitial lubrication theory is grounded in the fact that when loaded, the interstitial fluid of cartilage undergoes pressurization, and this pressurized fluid preferentially supports the applied stresses; resulting in a high fluid load support levels and low interfacial friction coefficients. When MCA configurations are present, high fluid load fractions and low friction coefficients can be supported during sliding near-indefinitely, provided the semi-spherical MCA probe/contact moves across the cartilage surface faster than the fluid in the underlying cartilage can depressurize (flow). By preventing exudation of fluid from cartilage, the MCA effectively shifts applied stresses away from the tissue’s solid matrix and onto the fluid phase, thereby promoting high lubricity and wear protection in cartilage
11. MCA contacts exist within articular joints, so they can contribute to joint function; however, they are unable to explain the full behavior of normal joints.
The SCA is a configuration whose usage predates that of the MCA
6,7,16. In the SCA, a small diameter (Ø ≤ 6mm) cartilage explant is pressed against a flat surface, typically glass or steel (Figure 1, top left). This counterface is then slid across the cartilage surface (reciprocally or unidirectionally
17,18, depending on the device being used) to assess frictional behaviors. SCA-based studies are ubiquitous within the cartilage lubrication field because the SCA creates a flat-on-flat contact between the cartilage and the compressing surface, enabling constant application of stress and facile assessment of deformation and friction. Upon initial compression of SCA contacts, interstitial fluid is pressurized within the tissue, temporarily shielding the matrix from stress and promoting low friction initially, in accordance with interstitial lubrication theory. However, load-induced fluid pressurization drives relatively rapid exudation of interstitial fluid into the low-pressure surrounding bath. This volumetric fluid loss leads to thinning of the tissue and a shift in load support from cartilage’s fluid phase to its solid phase, resulting in time dependent increases in friction during sliding. The ability to fully de-pressurized the SCA, and thereby eliminate interstitial lubrication, has been a crucial tool in studying the role of
boundary lubricants10,19–23 in cartilage tribology (Figure 1, bottom left). However, the SCA contact, and the conditions under which it operates, is a fairly poor representation of the normal joint environment.
Figure 1. Comparisons between the SCA, MCA, and cSCA highlight how these configurations can be used to investigate different lubrication regimes including boundary lubrication, sustained interstitial lubrication, and tribological rehydration.
Thus, despite, hundreds of studies utilizing both MCA and SCA testing configurations to study cartilage tribology over a period of decades, our field unfortunately remains largely ignorant of the many complexities of the tissue, as well as how cartilage actually manages to resist wear so well. If we take a step back, and look at the motion of the natural joint
in vivo, one appreciates that cartilage does not articulate solely as a flat-on-flat contact, nor as a migrating contact. Instead, the joint has a complex geometry, and joint articulation results in the production of complex motion patterns that includes stationary contacts, migrating contacts, high-speed sliding, bath exposure, loading and unloading, and the presence of sedentary (inactive) and active (articulating/sliding) periods. As a result, while both the MCA and the SCA have been quite informative in the development of individual cartilage lubrication theories, neither on its own can fully support the development of a unifying structure (or theory) of cartilage’s
in vivo lubrication behavior.
Our group, in collaboration with Dr. David Burris’s Materials Tribology Lab (also at the University of Delaware), has begun to leverage a newly rediscovered and slightly modified version of the SCA, called the convergent SCA (cSCA)
18,24–29 (Figure 1, top right), to uncover cartilage tribomechanical behaviors that shed light on a potential unifying theoretical lubrication structure. Like the SCA, the cSCA utilizes a flat counter-surface to apply compressive loads and slide across a cartilage explant. However, unlike the SCA, the cSCA utilizes much larger and convex-surfaced explants (e.g. Ø ≥ 12mm in bovine femoral condyle cartilage). The natural curvature of cartilage in these cSCA contacts generates a curved-on-flat contact between the cartilage and the compressing surface, promoting the formation of a
convergent wedge at the edges of contact that can contribute, via hydrodynamics, to the tribomechanical response of the tissue during sliding. Importantly, under sedentary loading conditions (sliding speed = 0 mm/s), the cSCA responds identically to the classical SCA in accordance with biphasic cartilage theory. However, if the cSCA is slid fast enough (e.g. at speed those of mimicking joint motion, 80-150 mm/s), fluid appears to be pumped back into the cSCA cartilage; halting, reversing, and recovering the loss of tissue hydration and interstitial fluid lost (i.e. exuded) as a result of compressive loading. We have termed this process
tribological rehydration24 (Figure 1, bottom right), and we have hypothesized that high-speed sliding against our explants drives hydrodynamics pressurization of fluid entrained into the converging wedges formed at the leading edge of cSCA contacts. This pressurized fluid then contributes to the recovery of fluid into the contact, which is partially ‘dehydrated’ by load-driven exudation; such competitive recovery of fluid leads to the ‘rehydration’ of the cartilage, recovery of interstitial fluid and pressures, tissue strain, and the promotion of low friction due to the restoration of high fluid load support and the sustenance of interstitial lubrication. This phenomenon also drives a powerful, and previously unappreciated, recovery of tissue lubricity.
While tribological rehydration was not necessarily anticipated by any single existing cartilage lubrication theory, its discovery is at least partially supported by the synthesis of aspects of existing theories (e.g. hydrodynamics/fluid film theory, interstitial lubrication theory, and bath exposure theory); unfortunately, these theories have been classically approached in a mutually exclusive manner allowing critical connections between them to be missed. Conversely, the cSCA has, in a very short while, presented itself as an ideal platform for initiating the long-sought unification of previously disparate and oft thought exclusive lubrication theories into a single lubrication structure capable of explaining the phenomenal friction and wear resistance observed for cartilage
in vivo. For example, the cSCA enables the typical stress-relaxation behavior of biphasic cartilage during sedentary behavior, while enhancing fluid load support and interstitial lubrication during active sliding. This produces both a physiological strain and friction response within a benchtop testing platform, a feat that had been impossible with other testing configurations
18,24–29.
Despite this new appreciation regarding cartilage function, a number of important questions regarding both basic cartilage tribology and translational tribology remain. For example, how do various activity patterns influence cartilage tribology and biomechanics (e.g. more frequent vs. less frequent bouts of articulation/sliding, shorter vs. longer durations of activity/sliding)? How do tissue-level injuries (i.e. fissures and defects, as opposed to joint-level injuries) affect both the immediate longer-term tribomechanics of tissue within this more physiologically-relevant benchtop testing system? How do boundary lubricants, either synthetic or natural, influence the friction and strain response of cartilage explants in the cSCA? And, can we use the cSCA as a platform to transform the study and our understanding of both disease-initiating changes and tissue longevity-promoting inputs to influence the physiological function and health of articular cartilage? The remainder of this article describes a selection of studies conducted within the Price and Burris labs to answer these important and clinically-relevant cartilage lubrication questions, as well as describe some of the fundamental connections that have been made in support of a grand unifying structure of lubrication theory.
II. The cSCA as a repeatable and reliable testing platform for investigating cartilage’s tribomechanical response to injury.
Having uncovered a number of unique behaviors of cSCA explants under high-speed sliding, we next wished to evaluate the influence of cartilage damage/injury on cSCA behavior and tribological rehydration. However, in advance of such a study, I had to convince myself of the robustness of the cSCA testing platform, and demonstrate its ability to be used to reproducibly perform repeated tribology tests on cartilage explants (data published)
29.
For these studies, I developed an abbreviated tribological rehydration characterization protocol, consisting of 30-minutes of static compression under an initial 7N (~0.3 MPa stress) load, followed by 30-minutes of reciprocal sliding (at 80 mm/s) under the same load. Each bovine cartilage explant (n = 8) was tested twice on the day of extraction (at 0- & 2-hours), and then daily for 4 more days. Explants were visually assessed prior to and after each test; repeatedly tested explants did not incur obvious damage or wear. Quantitatively, despite significant variability in sliding-driven strain recovery and equilibrium (end-of-sliding) friction coefficients among individual explants, neither outcome changed significantly from test-to-test or day-to-day for a given explant (Figure 2). This work illustrated the ability to repeatedly perform cSCA tribology tests on cartilage explants over the moderate-term (i.e. 5-days) without fear of markedly changing the tissue’s tribomechanical response or tribological rehydration ability.
Figure 2. Repeated tribological characterization confirmed that repeated testing in the cSCA does not influence strain recovery or equilibrium friction coefficients for up to 6 consecutive tests over 5 days. Each symbol/color represents an individual explant tested.
This study supported my next investigation: identifying whether clinically-relevant injuries to cSCA cartilage affected the tissue’s tribomechanical response to sliding
29. Articular cartilage fissures
30, defects
31, and impact injuries
30,32 are known to accompany joint injuries such as dislocations, meniscal tears, and tendon ruptures, and may contribute to the increased risk of osteoarthritic disease development
8,9,32–34. Thus, we investigated the influence of i) full-thickness cartilage fissures (both parallel and perpendicular to the direction of sliding); ii) small (3mm) vs. large (6mm) chondral defects (both in the center of contact and edge of contact); and iii) mild (9-13 MPa) vs. moderate (15-40 MPa) vs. severe (45-65 MPa) compressive tissue impacts on the acute ability of cartilage to support sliding-driven tribological rehydration. Tribological characterization was performed prior to and again following the creation of each respective injury to facilitate pair-wise comparisons.
Our results demonstrated that the presence of physical damage to cartilage tissue acutely compromises tribological rehydration and the sliding-driven recovery of cartilage function in an injury-specific manner (Figure 3)
29. Cartilage injuries associated with full-thickness damage to the cSCA (i.e. linear fissures and chondral defects) immediately and markedly suppressed cartilage’s ability to support sliding-induced tribological rehydration and lubricity, suggesting that such injuries may compromise the tissue’s ability to maintain interstitial pressurization/lubrication through the creation of pathways by which pressurized fluid can escape the contact. Since such injuries acutely compromise tribological rehydration and the tribomechanical properties of the tissue, if present, they may place the long-term health of cartilage into immediate jeopardy. In contrast, injurious impacts, which caused more surface-limited damage to cSCA explants, had remarkably little effect on the tissue’s ability to successfully support sliding-induced tribological rehydration, possibly because such damage may be more easily ‘sealed off’ hydrodynamically from the convergent edges. This understanding of how injury alters cartilage tribomechanics mechanisms can guide future studies of the longer-term functional consequences of physical injury and joint articulation on cartilage health, disease, and rehabilitation.
Figure 3. Abbreviated injury results showing median strain recovery and equilibrium friction coefficient before and after injury for fissures (left), defects (center, where the center line in each group represents the small 3mm defect), and impacts (right).
III. Incorporation of physiologically relevant activity modeling within cSCA tests fundamentally altered our understanding of how cartilage works.
Our initial work regarding the discovery and characterization of tribological rehydration in the cSCA were founded upon studies utilizing continuous sliding. While these studies were powerful, they are not realistic because joint motion includes periods of inactivity (i.e. sitting, standing) and activity (i.e. walking, moving). Additionally, the loads that cartilage endures throughout a typical day changes with motion, and during rest or sleeping periods. Thus, we wished to investigate the influence of intermittent activity, i.e. cyclic activity and inactive/sedentary periods, on cartilage tribology in the cSCA. This is especially relevant given the general decreases in physical activity associated with modern lifestyles and recently identified connections between inactivity and morbidity/mortality.
Recent work from our team has begun to investigate the influence of physiologically-relevant activity patterning, i.e. those that contain intermittently applied activity, on the tribomechanical functions of cartilage
25,28,35. In order to accomplish this, a number of aspects of our benchtop sliding tests needed to be considered. First, how does one equivalently scale testing time between an
ex vivo test and a typical human day? Second, how much sliding time should one apply to model typical daily joint motions/activities? And lastly, how should one distribute intermittent sliding to model clinically-relevant activity paradigms? Due to size-scaling, the biomechanical response of ~Ø19mm cartilage explants are necessarily faster than that of cartilage in the intact joint. Comparing
in vivo human knee contact diameters and pressures to those of our cSCA samples (described previously)
28, allowed the scaling-down of a ‘typical’
in vivo day to a 150-minute cSCA testing block. Each of these simulated ‘days’ started with 90 minutes of ‘awake’ time under a 5N load (~0.25 MPa stress), followed by 60 minutes of ‘sleep’ time under a 0.1N load; allowing us to model, to a first approximation, diurnal average contact stresses experienced
in vivo. The CDC recommends that adults engage in ~30-minutes of moderate activity per day, so we interspersed 30-minutes of sliding activity across our ‘awake’ period, administering the sliding in 1, 3, 6, or 15 equally distributed bouts (Figure 4)
28. Thus, total sliding and inactive time was set at 30- and 60-minutes, respectively, and we focused on investigation of the influence of different activity distributions/frequencies on tissue function. Sliding was applied at 100 mm/s to replicate the sliding speeds of femoral condyle cartilage sliding past tibial cartilage during walking
28,35.
We found that the manner in which active and sedentary bouts were patterned, namely the total continuous time spent inactive during a single bout, had a major influence on cartilage tribomechanical function (Figure 4). Longer periods of inactivity (i.e. during tests with 1 bout of sliding [30-min]) led to higher peak strains, greater depressurization of the tissue, higher start-of-sliding frictions, and higher time-averaged frictions compared to tests with shorter periods of inactivity (i.e. during tests with 15 bouts of sliding [each 2-min long]), indicating that joints may actually benefit from increased, intermittent activity because more frequent joint motion can drive lower overall strains and frictions. That said, strain was recovered in all tests, regardless of the number of activity cycles applied during the awake period, indicating that active sliding supports tribological rehydration, regardless of the length of time sliding occurs. Furthermore, strain recovered during sliding was actually greater than the recovery that occurred during sleep, suggesting that cartilage may be unable to recover through rest alone, and that
activity is necessary for maintaining cartilage hydration. This work identified that we are capable of modulating cartilage strain and friction in the cSCA simply by altering activity patterns, and represent just the ‘tip of the iceberg’ regarding our understanding of the influence of activity structure on cartilage function; further findings will undoubtedly re-write our understanding of how activity can, somewhat counterintuitively, promote beneficial joint health outcomes.
Figure 4. Simulated day of sliding starting with an ‘awake’ period of 5N loading containing intermittent bouts of sliding at 100 mm/s and sedentary compression, followed by a ‘sleep’ period of 0.1N loading with no sliding (left). Different numbers of sliding bouts during the ‘awake’ period cause variable peak strains, but lead to similar recovery (center). Start-of-sliding friction increases with length of sedentary interval, however end-of-sliding friction remains low for all activity patterns (right). Figure adapted from Graham 2017 BMES Annual Meeting abstract35 and Graham 2020 CTR28.
IV. ‘Complicating’ the story with the addition of biological lubricants
Until this point, all of our cSCA studies had been performed in the presence of PBS, however,
in vivo, cartilage is bathed in synovial fluid (SF)
7,36–38. Synovial fluid is a viscous material containing hyaluronic acid (HA) at concentrations 1-5 mg/mL
22, and lubricin; classical SCA-based studies utilizing SF, and HA and lubricin, indicate that these materials have substantial boundary lubricant properties
7,36–38. In striving to explore the physiological-consistency of our benchtop cSCA testing platform we recently investigated the influence of the addition of bio-lubricants (SF & HA) on the strain and friction response of cSCA cartilage under high-speed sliding (
manuscript in preparation). We also undertook this study to establish bathing solution compositions with the ability to support ‘biologically safe’ friction coefficients long-term (μ < 0.05) during benchtop sliding tests.
In these studies, bovine cartilage explants were subjected to our 60-minute tribological characterization protocol first in the presence of PBS to establish the a baseline tribological behavior, then again after free-swelling (and sliding) in the presence of lubricant. Lubricants tested included equine SF, 5 mg/mL HA, PBS + bovine serum albumin (BSA), and chondrogenic culture media (CCM); solutions used commonly used in explant materials testing and culture studies
25,27,39,40. Focusing on strain recovery and equilibrium friction, we saw that SF and HA
increased strain recovery and markedly
decreased equilibrium friction during tribological characterization. Notably, the presence of SF and HA during sliding caused a ~10-fold reduction in equilibrium friction coefficient compared to their paired PBS controls, from an impressive μ ≈ 0.02 to an unprecedented μ ≈ 0.003-0.004 (Table 1). BSA and CCM did not improve (or hinder, compared to PBS) the tribological response of cartilage to sliding; however, sliding in PBS, BSA, and CCM still resulted in impressive and ‘physiologically-safe’ equilibrium friction behaviors.
Table 1. Equilibrium friction coefficients measured at the end of sliding show significant decreases in explants tested with SF and HA compared to baseline PBS behavior.
Figure 5. Pair-wise testing was performed on cSCA explants to identify the influence sliding in the presence of synovial fluid (SF), hyaluronic acid (HA), bovine serum albumin (BSA), and chondrogenic culture media (CCM) all compared to baseline sliding in the presence of phosphate-buffered saline (PBS).
This study is critical in that it has demonstrated the ability of the cSCA platform to greatly expand the functional state-space over which cartilage tribomechanics can be interrogated (Figure 6). Much of our early understanding of cartilage tribomechanics and chondrocyte mechanobiology has been derived from classical SCA tests, which can only support long-term sliding tests at relatively ‘low’ ‘semi-physiological’ friction coefficients (μ ≈ 0.05)
17,32 through the use of boundary lubricants; ‘non-lubricated’ SCA tests only support ‘pathological’ long-term frictional outcomes (μ > 0.3)
17,24,32, as a result of complete loss of interstitial lubrication. When slid at slow speeds, the cSCA is capable of generating similiar
pathological and
semi-physiological tribological environments
18,24–29. However, under high-speed sliding, the cSCA is uniquely suited to support tribology testing under a range of semi-physiological (μ ≈ 0.02
24,27,29, in the absence of lubricants) to truly
physiological frictional conditions (μ ≈ 0.002-0.02), in the presence of lubricants, (
unpublished data).
Figure 6. New state-space of cartilage benchtop testing, showing our ability to achieve truly physiological friction coefficients while sliding in the cSCA.
The cSCA thus represents a unique platform for exploring cartilage’s true physiological function due to its unprecedented ability to support and sustain tribomechanical behaviors that closely match those seen
in vivo through the presence of tribological rehydration. Furthermore, the precise manipulability of the cSCA provides us with a single benchtop testing configuration that can interrogate the full breadth of strain and friction states that cartilage may encounter, providing a transformative tool to better understand cartilage’s phenomenal tribological properties and wear resistance and to support the creation of a unifying theory of cartilage lubrication.
V. Improved Physiological-Relevance allows the Investigation of Processes Associated with Cartilage Homeostasis, and Disease Initiation and Progression
Ultimately, the goal of my research is to use the cSCA platform to investigate not only cartilage tribomechanics, but to better inform our understanding of cartilage mechanobiology (the biological response of the tissue) in response to the strains and stresses encountered during typical joint motion. The cSCA platform has enabled me to subject live, metabolically active cartilage explants to a range of physiologically-relevant activity regimes and sliding conditions, and quantify both the tribological and biological responses of cartilage to mechanical stressors thought to be involved in osteoarthritis and joint disease initiation/progression (
experiments in progress).
I am currently using the ability to precisely titrate both strain and friction in the cSCA to identify primary tribomechanical drivers of cell death in healthy equine articular cartilage (Table 2). By utilizing tribological testing patterns with different sedentary compression and sliding behaviors, e.g. immediate vs. delayed sliding, fast (80 mm/s) vs. slow (1 mm/s) sliding speeds, with and without SF as a lubricant) I have begun to identify the specific contributions of tissue strain and friction to chondrocyte cell death.
Table 2. Groups for decoupling strain and friction to evaluate cartilage’s biological response to sliding in the cSCA.
Performing tests with difference combinations of sedentary compression, sliding speeds, and lubricants has allowed us to de-couple strain and friction to begin to identify whether friction or strain is a primary driver of cell death. Each explant remains in culture for 2 hours after tribology testing to allow the cells within the tissue to respond, prior to imaging with stains that identify live vs. dead cells. Upon completion of this study, we hope to identify the roles strain and friction play in explant cell death in the cSCA, which we can use to drive future work investigating the moderate- to long-term consequences of both on not only healthy cartilage but also injured/pre-osteoarthritic cartilage.
VI. Summary
In conclusion, the cSCA has reshaped our ability to perform cartilage benchtop testing in a manner that is both physiologically-relevant and exquisitely manipulatable. This testing configuration has uncovered a lubrication mechanism, tribological rehydration, that we can potentially use to build a unifying structure of cartilage lubrication off of. The newly rediscovered cSCA configuration also represent a uniquely transformative tool for conducting transformative cartilage tribology and mechanobiology study, which will ultimately allow us to answer crucial questions regarding the behavior and resiliency of healthy cartilage and the processes involved with arthritic disease initiation and progression.
VII. Acknowledgements
I’d like to thank the Society of Tribologists and Lubrication Engineers for their assistance and support in my tribological studies. I’d also like to thank Dr. Kyla Ortved from the University of Pennsylvania School of Veterinary Medicine for her assistance in both idea generation and obtaining live equine cartilage and healthy synovial fluid for the use in these studies. This material is based upon work supported by Society of Tribologists and Lubrication Engineers Elmer E. Klaus Fellowship as well as the National Science Foundation (NSF) Biomaterials and Mechanobiology program [1635536] and the NSF Graduate Research Fellowship Program [1247394]. Any opinions, findings, and conclusions or recommendations expressed in this material are those of the authors and do not necessarily reflect the views of the National Science Foundation.
VIII. References
1.
MacConaill, M. a. The Function of Intra-Articular Fibrocartilages, with Special Reference to the Knee and Inferior Radio-Ulnar Joints.
J. Anat. 66, 210–227 (1932).
2.
Jones, E. S. Joint Lubrication.
Lancet 227, 1043–1045 (1936).
3.
Ateshian, G. A. & Hung, C. T. The natural synovial joint: properties of cartilage.
IMechE 220, 657–670 (2006).
4.
Armstrong, C. G., Lai, W. M. & Mow, V. C. An Analysis of the Unconfined Compression of Articular Cartilage.
J. Biomed. Eng. 106, 165–173 (1984).
5.
Mow, V. C., Holmes, M. H. & Michael Lai, W. Fluid transport and mechanical properties of articular cartilage: A review.
Journal of Biomechanics (1984). doi:10.1016/0021-9290(84)90031-9
6.
Rajan, V., Caligaris, M., Hung, C. T., Ahmad, C. S., & Ateshian, G. A. Hemiarthroplasties defeat interstitial fluid pressurization in cartilage and promote greater friction than natural joints.
Annu. Meet. Orthop. Res. Soc. 2120, (2010).
7.
Forster, H. & Fisher, J. The influence of loading time and lubricant on the friction of articular cartilage.
Proc. Inst. Mech. Eng. Part H J. Eng. Med. 210, 109–118 (1996).
8.
Pawaskar, S. S., Jin, Z. M. & Fisher, J. Modelling of fluid support inside articular cartilage during sliding.
Proc. Inst. Mech. Eng. Part J-Journal Eng. Tribol. 221, 165–174 (2007).
9.
McCutchen, C. W. The Frictional Properties of Animal Joints.
Wear 5, 1–17 (1962).
10.
Caligaris, M. & Ateshian, G. A. Effects of sustained interstitial fluid pressurization under migrating contact area, and boundary lubrication by synovial fluid, on cartilage friction.
Osteoarthr. Cartil. 16, 1220–1227 (2008).
11.
Moore, A. C., Schrader, J. L., Ulvila, J. J. & Burris, D. L. A review of methods to study hydration effects on cartilage friction.
Tribol. - Mater. Surfaces Interfaces 11, 202–214 (2017).
12.
Krishnan, R., Kopacz, M. & Ateshian, G. A. Experimental verification of the role of interstitial fluid pressurization in cartilage lubrication.
J. Orthop. Res. 22, 565–570 (2004).
13.
Ateshian, G. A., Wang, H. Q. & Lai, W. M. The role of interstitial fluid pressurization and surface porosities on the boundary friction of articular cartilage.
J. Tribol. Asme 120, 241–248 (1998).
14.
Ateshian, G. A. The role of interstitial fluid pressurization in articular cartilage lubrication.
J. Biomech. 42, 1163–1176 (2009).
15.
Moore, A. C. & Burris, D. L. An analytical model to predict interstitial lubrication of cartilage in migrating contact areas.
J. Biomech. 47, 148–153 (2014).
16.
Wright, V. & Dowson, D. Lubrication and cartilage.
J. Anat. 121, 107–18 (1976).
17.
Bonnevie, E. D. et al. Microscale frictional strains determine chondrocyte fate in loaded cartilage.
J. Biomech. 74, 72–78 (2018).
18.
Burris, D. L., Ramsey, L., Graham, B. T., Price, C. & Moore, A. C. How Sliding and Hydrodynamics Contribute to Articular Cartilage Fluid and Lubrication Recovery.
Tribol. Lett. 67, 1–10 (2019).
19.
Reimann, I. Pathological Human Synovial Fluids. Viscosity and Boundary Lubricating Properties.
Clin. Orthop. Relat. Res. 119, (1976).
20.
Elsaid, K. A., Jay, G. D., Warman, M. L., Rhee, D. K. & Chichester, C. O. Association of articular cartilage degradation and loss of boundary-lubricating ability of synovial fluid following injury and inflammatory arthritis.
Arthritis Rheum. 52, 1746–1755 (2005).
21.
Bell, C. J., Ingham, E. & Fisher, J. Influence of hyaluronic acid on the time-dependent friction response of articular cartilage under different conditions.
Proc. Inst. Mech. Eng. Part H J. Eng. Med. 220, 23–31 (2005).
22.
Schmidt, T. A., Gastelum, N. S., Nguyen, Q. T., Schumacher, B. L. & Sah, R. L. Boundary Lubrication of Articular Cartilage: Role of Synovial Fluid Constituents.
Arthritis Rheum. 56, 882–891 (2007).
23.
Bonnevie, E. D., Galesso, D., Secchieri, C. & Bonassar, L. J. Degradation Alters the Lubrication of Articular Cartilage by High Viscosity , Hyaluronic Acid-Based Lubricants.
J. Orthop. Res. 1456–1464 (2018). doi:10.1002/jor.23782
24.
Moore, A. C. & Burris, D. L. Tribological rehydration of cartilage and its potential role in preserving joint health.
Osteoarthr. Cartil. 25, 99–107 (2017).
25.
Graham, B. T., Moore, A. C., Burris, D. L. & Price, C. Sliding enhances fluid and solute transport into buried articular cartilage contacts.
Osteoarthr. Cartil. 1–8 (2017). doi:10.1016/j.joca.2017.08.014
26.
Burris, D. L. & Moore, A. C. Cartilage and Joint Lubrication: New Insights Into the Role of Hydrodynamics.
Biotribology 12, 8–14 (2017).
27.
Graham, B. T., Moore, A. C., Burris, D. L. & Price, C. Mapping the spatiotemporal evolution of solute transport in articular cartilage explants reveals how cartilage recovers fluid within the contact area during sliding.
J. Biomech. 1–6 (2018). doi:10.1016/j.jbiomech.2018.01.041
28.
Graham, B. T., Moore, A. C., Burris, D. L. & Price, C. Intermittent Activity and the Biomechanical Response of Cartilage to Sliding: Detrimental Effects of Long Sedentary Bouts.
Connect. Tissue Res. (2020).
29.
Farnham, M. S., Larson, R. E., Burris, D. L. & Price, C. Effects of mechanical injury on the tribological rehydration and lubrication of articular cartilage.
J. Mech. Behav. Biomed. Mater. 101, 551–556 (2020).
30.
Ewers, B. J., Dvoracek-Driksna, D., Orth, M. W. & Haut, R. C. The extent of matrix damage and chondrocyte death in mechanically traumatized ...
J. Orthop. Res. 19, 779–784 (2001).
31.
Falah, M., Nierenberg, G., Soudry, M., Hayden, M. & Volpin, G. Treatment of articular cartilage lesions of the knee.
Int. Orthop. 34, 621–630 (2010).
32.
Bonnevie, E. D. et al. Sub-Critical Impact Inhibits the Lubricating Mechanisms of Articular Cartilage.
J. Biomech. (2017). doi:10.1016/j.jbiomech.2016.12.034
33.
Mansour, J. M. Biomechanics of Cartilage. in
Kinesiology: the mechanics and pathomechanics of human movement 66–79 (Philadelphia, PA: Lippincott Williams and Wilkins, 2003). doi:10.1002/art.23548
34.
Dabiri, Y. & Li, L. Focal cartilage defect compromises fluid-pressure dependent load support in the knee joint.
Int. j. numer. method. biomed. eng. 31, (2015).
35.
Graham, B. T., Moore, A. C., Burris, D. L. & Price, C. Mitigating Articular Cartilage Strains through Regular Activity.
BMES Annu. Meet. (2017).
36.
Bonnevie, E. D., Puetzer, J. L. & Bonassar, L. J. Enhanced boundary lubrication properties of engineered menisci by lubricin localization with insulin-like growth factor I treatment.
J. Biomech. 47, 213–2188 (2014).
37.
Hou, J. S., Holmes, M. H., Lai, W. M. & Mow, V. C. Boundary Conditions at the Cartilage-Synovial Fluid Interface for Joint Lubrication and Theoretical Verifications.
J. Biomech. Eng. 111, 78 (1989).
38.
Linn, F. C. Lubrication of animal joints.
J. Bone Jt. Surg. (1967).
39.
Bush, P. G. & Hall, A. C. The osmotic sensitivity of isolated and in situ bovine articular chondrocytes.
J. Orthop. Res. 19, 768–778 (2001).
40.
Durney, K. M. et al. Physiologic Medium Maintains the Homeostasis of Immature Bovine Articular Cartilage Explants in Long-Term Culture.
J. Biomed. Eng. 141, (2019).