Tribological Bearing Testing
Jeanna Van Rensselar, Contributing Editor | TLT Feature Article April 2014
Modern technology has improved (but not perfected) our ability to predict failure for these critically important components.
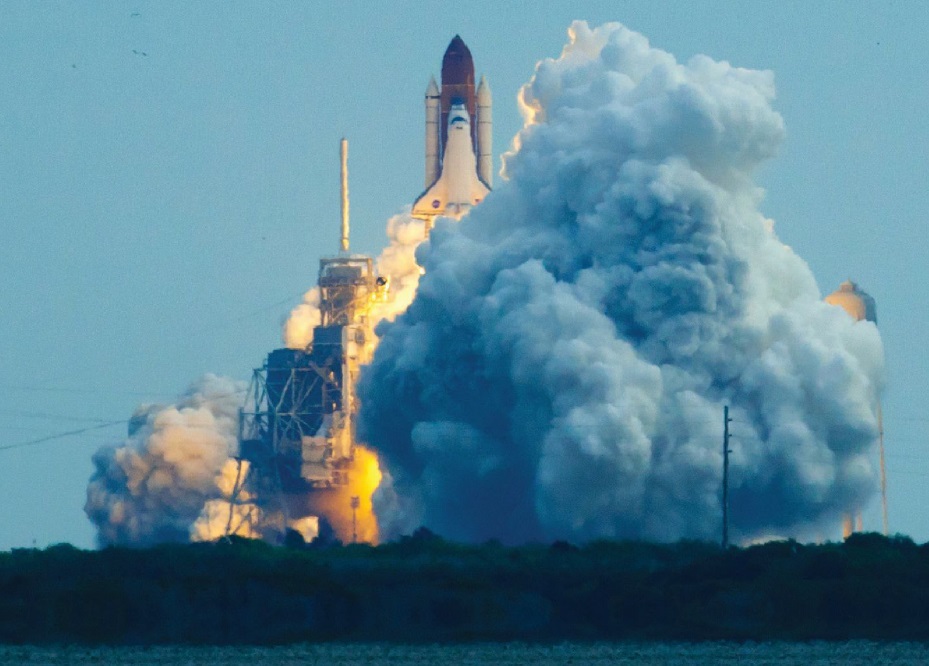
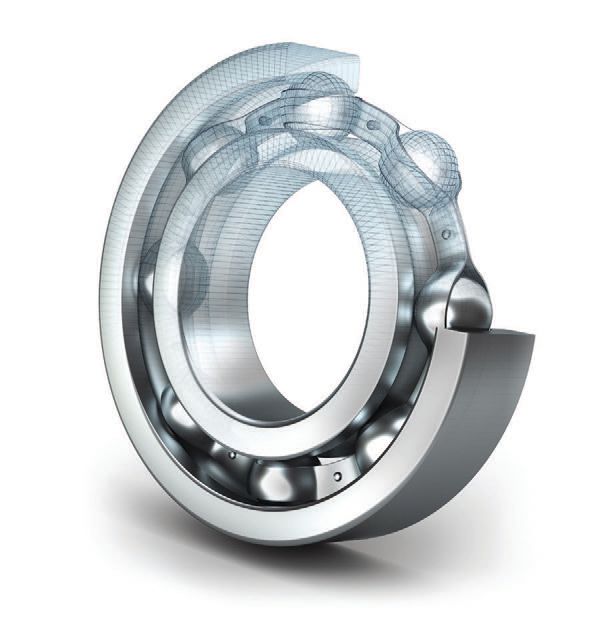
www.canstockphoto.com
KEY CONCEPTS
•
With modern improvements in manufacturing, testing technology and care, bearing life has been extended 400 fold since the 1940s.
•
The bearing fatigue limit incorporated into ISO Standard 281:2007 is not universally accepted.
•
Of the three bearing test categories, no one test is ideal.
THE WORLD’S SMALLEST BEARINGS have an inner diameter of 0.5 mm, an outer diameter of 1.5 mm and a width of 0.65 mm (about the size of a pinhead). They are used in devices that require extreme precision on a nano scale, such as miniaturized medical devices and micro-motors.
At the other extreme, some bearings measure 18 meters on their outer diameter and weigh more than 15 tons. These are used in giant tunnel boring machines.
In between are a multitude of machines that rely on the accuracy and durability of their bearings.
A space shuttle is propelled into orbit by two solid rocket motors and three liquid-fed main engines. After the solid motors fall away, the shuttle engines continue to run for eight minutes. During this time, low- and high-pressure turbo pumps inject the engines with fuel. A critical component of the turbo pump is the main shaft, which supports the drive turbine, pump inducer and impeller. During rotation, rolling element bearings hold the shaft in place. If the bearings were to fail, the shaft would move out of position, creating physical contact between the turbo pump components in a fuel-rich environment. The result could be catastrophic (
1).
According to STLE-fellow Erwin V. Zaretsky, P.E., Consulting Engineer, Distinguished Research Associate, NASA Glenn Research Center in Cleveland, Adjunct Professor of Engineering, Case Western Reserve University (one of the top practitioners in the field), the design and development of the space shuttle turbo pump bearings evolved over several decades. They were based on computer analysis, laboratory rig testing and static ground testing of the shuttle turbo pumps under simulated flight conditions. The bearing computer analysis alone could not predict with reasonable engineering certainty the endurance and failure characteristics of these bearings.
The most thorough test (application simulation) is too time-consuming and costly for most bearing manufacturers and OEMs. And some experts question the validity of computer simulation and bench tests that analyze bearings in isolation.
The condition that most often limits bearing function and longevity is rolling element fatigue and the most common predictability calculation of failure is
L10 bearing life. This method was first proposed in 1924 by Swedish researcher Arvid Palmgren. The
L10 bearing life (the point in hours or bearing inner-ring revolutions at or before, which 10 percent of the bearings in a group will have failed by rolling element fatigue), is based on Palmgren’s observation that no bearings in a group run under the same conditions or fail at the same time (
2). In other words, bearing life is probabilistic or distributive, not deterministic.
L10 BEARING LIFE CALCULATION
The
L10 rating life of a group of identical roller bearings is the number of revolutions that 90 percent of bearings in a group will complete or exceed before the first evidence of fatigue (the point at which exactly 10 percent of the bearings are showing signs of fatigue).
L10 is calculated in terms of millions of revolutions or in terms of hours.
Rolling element fatigue is a spall manifesting itself across the width of the running track and through to the depth of the maximum shearing stress beneath the contact surface. A spall can begin as a crack from a subsurface inclusion, defect or void below the contacting surface or from a crack emanating from a surface defect or a debris dent that spreads into a network of cracks.
For 90 to 95 percent of machine design applications, the recommendations in bearing manufacturers’ catalogs lead to safe and reliable functioning. Remaining applications require specialized knowledge and analysis (
3).
Rolling element fatigue occurs in both bearing races and rolling elements. It is extremely variable but statistically predictable depending on such life factors as:
•
Operating conditions
•
The method of steel processing
•
The method of bearing manufacture (including the heat treatment)
•
The steel type
•
The lubricant.
Fatigue failures that originate below the contacting surface (classic rolling element fatigue) are an inevitable result of age. But most bearings are removed for other reasons before this happens.
Failures other than those caused by age (rolling element fatigue) can be avoided as long as the bearing is not overloaded and is correctly designed, installed, lubricated and not subject to harsh operating conditions. With improvements in manufacturing, today’s testing technology and care, bearing life has been extended appreciably.
The term
basic bearing life refers to the
L10 life without dependent life factors such as those listed above. Because the vast majority of undamaged bearings are removed from service for reasons other than the end of their calculated
L10 life, it is cost-effective to inspect and place back into service those undamaged bearings that were removed before reaching their
L10 life.
Per Zaretsky, probable causes for rolling element bearing removal and the approximate percent of failures include:
•
Fatigue (surface and subsurface) (3 percent)
•
Cage wear (3 percent)
•
Wear (6 percent)
•
Handling damage (7 percent)
•
Dimensional discrepancies (17 percent)
•
Debris denting/contamination (20 percent)
•
Corrosion pitting (27 percent)
•
Other (17 percent).
Other causes include true and false brinelling, misalignment, bearing overload, excessive thrust lubrication, roller-edge stress, electric arc discharge and cage element or ring fracture. While these causes can be mitigated, they can never be completely eliminated. This makes understanding and determining bearing life even more important.
ISO 281:2007
ISO 281:2007 specifies methods of calculating the basic dynamic load rating of roller bearings manufactured from high-quality hardened bearing steel. It also specifies the methods of calculating the basic rating life (
L10). In addition, ISO 281:2007 specifies methods of calculating the modified rating life; where lubrication condition, lubricant contamination, fatigue load of the bearing and other factors are taken into account. It does not cover the effects of wear, corrosion and electrical erosion on bearing life. ISO 281:2007 does not apply to designs where the rolling elements operate directly on a shaft or housing surface, unless that surface is equivalent in all respects to the bearing ring (or washer) raceway it replaces.
BEARING TESTS
There are three basic types of bearing tests: application simulation, computer simulation and individual bearing tests in the lab. Each method has its pros and cons. Design engineers tasked with bearing acceptance testing need to determine which test(s) best satisfies their specific situation.
STLE-member Joe Braza, senior scientist for Lancer Systems in Allentown, Pa., explains, “For plain bearings, there are straightforward service life equations that can be used to predict the life of a bearing. These equations depend on a number of factors such as load, speed, lubrication, temperature, dimension (i.e., radial clearance) and material, including understanding the effect of contamination or seal design. In developing new plain bearing materials, these factors are not yet defined; therefore, bearing testing needs to occur before any type of bearing life formulas can be used.”
Let’s examine the three bearing-test categories.
Application Simulation. For some OEMs, there is no substitute for actual application simulation testing. During this type of analysis, bearings are tested in either a prototype or the actual assembly where they operate. For complex simulations, the bearing manufacturer and OEM may need to develop a formal test plan together. In some less-critical machinery, one way around application simulation is for the bearing manufacturer to supply bearings in mounted assemblies.
Application simulation tests ensure that the bearings will perform as specified in actual application operating conditions. While this is true, application simulation is very expensive in terms of time and dollars and yields comparatively paltry statistics.
Actual application testing is more important in some bearing applications than others—especially where there is no room for failure. Examples are wind turbines, where post-installation repairs are extremely costly; spacecraft, where repairs are either costly or impossible; and CT scanners where a noisy bearing makes the machine effectively unusable. Application testing makes sense just about any place where bearings are key to the proper functioning of critical equipment.
Actual bearing performance can stray from computer simulation predictions because of unknown load and mounting effects (incomplete input data) and variations in tolerances. While bearing inspections verify physical characteristics, they don’t provide any information about performance characteristics like torque and effective operating tolerances. Application testing resolves these issues.
“Optimal testing should replicate the application as closely as possible in terms of load, speed, temperature, lubrication and environment,” Braza says. “Besides closely simulating the application, the bearing tester should be designed with fixture flexibility to accommodate various test coupons, force transducers or measuring devices (load cells, thermocouples, speed sensors and accelerometers) and data acquisition system to provide information about the bearing performance—particularly in real-time.”
Braza adds, “One word of caution. The majority of the time researchers design accelerated tests to speed up the process of obtaining bearing information quickly. The downfall of accelerated testing is that the bearing may fail by a different mechanism and not be representative of the actual service condition.”
Computer Simulation. If performed carefully, computer simulation provides the answers to real-world functionality. It is a good alternative to bearing tests and application simulations where those costs would be prohibitive or the scale would be unwieldy or the logistics just too complex. Another good reason to opt for computer simulation is security.
But the accuracy of computer simulations depends on the validity of the simulation models and the consistency of the results. The simulation model should provide the same (or nearly the same) result for each execution. The process is much easier if the equations for simulation are already in place. Accurate calibration, verification and validation of simulation models are the keys to success. Tribologists are advised to understand these three factors.
1. Calibration. The base model’s parameters should be specified and calibrated so that the model matches the ultimate application as closely as possible. Of the three types of errors that can affect calibration (input error, model error and parameter error), input error and parameter error can be remedied by the user. But since model error is rooted in the methodology, it requires more to fix. Another consideration is that simulation models can produce conflicting results if they are based on different modeling theories.
2. Verification. Once calibrated, the model must be verified to ensure that it is operating as expected based on the statistical input. Verification is achieved by comparing initial output data with what is expected from the input data. Basically it is an analysis of the output to see if it is reasonable. For example, in bearing simulation, the type and level of damage can be verified to ensure that it is reasonably close to what the researcher would expect, given the parameters.
3. Validation. Once the model has been verified, the final step is to validate it by statistically comparing the outputs to existing historical data. This establishes the model’s ability to replicate reality. The process of validation highlights the importance of careful planning, thoroughness and accuracy during calibration and verification.
Computer simulations can be time and money-saving substitutes for bearing tests and lab simulations, but in order to be relevant they must accurately model the intended use. Unless these techniques are employed, the accuracy of the model will always be open to question.
“No matter how good the bearing computer analysis is, the successful operation of the system is dependent on the boundary conditions and assumptions that are inserted into the computer analysis,” Zaretsky says. “The difference in performance between the predicted and the experimental results can mean the difference between a successful product and a financial disaster.”
He continues, “In the last four decades, bearing modeling and analysis based on theoretical analysis has become very sophisticated and reasonably accurate in predicting bearing performance, life and reliability based on classical rolling element fatigue. There are 26 variables that can affect rolling element fatigue (
4). However, some of these variables are not necessarily susceptible to accurate analysis. As a result, testing rather than computer analysis may be a condition precedent for reliable bearing operation for some critical applications.”
Bearing Tests in the Lab. STLE-member Harvey Nixon, senior technologist, bearings, for Meritor Heavy Vehicle Systems in Troy, Mich., says, “Laboratory testing plays a rather large role in determining the design and manufacturing capability of the supplier. Such testing is done at both the component level (individual bearings) and in the intended application positions.”
Napoleon Engineering Services (NES) in St. Olean, N.Y., operates the largest independent bearing test facility in the U.S. Its bearing test lab programs focus on three main aspects of testing: life (dynamic), environmental and impact/static testing.
Life (dynamic) Testing. For OEMs, laboratory bearing life testing is performed as a means of comparing the performance of multiple suppliers. The most cost-effective method is via the standard bearing test (SBT) for validation of bearing design, material quality, manufacturing capability and overall workmanship quality. An SBT is performed without significant input on application conditions.
Considerations include application loads, speeds and lubricant conditions, but the test is accelerated to shorten overall test time—with considerable effort to maintain a failure mode common to the application. The tester runs the bearings to the point of failure, using classical or sudden-death testing methods. This yields relatively large quantities of data.
The lab generates a Weibull plot from the failure times (or failure and suspension times), and the result is an empirically derived supplier reliability metric. The addition of upper and lower confidence boundaries establishes the anticipated variability within a supplier’s population. When results between suppliers are compared, they provide a valuable understanding of relative performance differences. Lab testing of a baseline bearing supplier with known application experience allows an OEM to predict expected bearing life in an application from a new supplier.
Although SBT is the most common and cost-effective bearing test, it is also possible to perform theoretical application simulation (TAS) in the lab. The difference between TAS and SBT is that TAS test conditions are application- driven. Bearing loads and speeds are representative of how a bearing will perform within a particular application. Duty-cycle testing falls into this category. As a result, TAS tests often take longer to reach failure or are run only to a suspension point. The testing benefit to the OEM is the knowledge and confidence that they have tested the bearing under simulated real-life performance conditions. However, results are based on a smaller data subset.
Dynamic lab testing can have the added benefit of yielding additional test results without significantly influencing a test budget and lead time. Examples include:
•
Supplier life adjustment factors
•
Catalog load rating validation
•
Bearing efficiency comparison – torque and temperature
•
Weakest link failure mode – due to design or quality of workmanship
•
Model correlation
•
Material/process validation.
Environmental Testing. When primary bearing failure is due to external contamination (rather than pure fatigue failure), the ability of the seal to withstand contamination intrusion becomes paramount. Environmental lab tests that use mud slurry, water spray and fine particle dust are the tests of choice because of their relatively short runtimes and ability to replicate harsh operating environments. The end result is significant time savings over application-style field testing for comparing seal efficiency (
5).
Impact/Static Load Testing. Due to the increase in the global supply of through-hardened bearing materials, over case-carburized steel in tapered roller bearings (TRB) and mast guide bearings, impact and static load lab testing is on the rise. Such testing provides information on cone rib flange resistance to fracture on TRBs and true-brinelling indentation depth for mast guide bearings. Simple test fixtures can create extreme load and impact conditions without requirements for full application accessories to create the failure condition.
By using test rigs and test parameters with relatively short test times and lower costs, standard bearing testing examines operational differences between suppliers or designs. But it does not take intended application conditions into consideration.
Nixon explains, “Bench testing of the individual bearings is used most often for validation after the bearing samples have met all the initial scrutiny in the multistep evaluation process. Bench testing can be less expensive than full-scale application testing, and more bearing samples can be evaluated in less time. The more test data, the better the reliability of the conclusions about the performance capability of the bearing products. By necessity, the loading is higher in order to accelerate the testing. It is then important to compare the results to the life performance prediction model and compare it to a known validated supplier performance baseline (the existing production supply base, i.e., comparative testing of two suppliers).
THE WEIBULL PLOT (6)
The Weibull plot is a graphical technique for determining if a dataset comes from a population that would work with a 2-parameter Weibull distribution (one of the most widely used lifetime distributions in reliability engineering). The Weibull Plot has scales that are designed so that the points will be linear (or nearly linear) as long as the dataset follows a Weibull distribution.
The least squares fit of this line yields estimates for the shape and scale parameters of the Weibull distribution. The shape parameter is the reciprocal of the slope of the fitted line and the scale parameter is the exponent of the intercept of the fitted line.
The Weibull distribution also has the property that the scale parameter falls at the 63.2 percent point regardless of the shape parameter value. The plot shows a horizontal line at this 63.2 percent point and a vertical line where the horizontal line intersects the least squares fitted line. This vertical line shows the value of scale parameter.
WHEN TO TEST
Bearing tests are necessary when the proper functioning of a critical or costly machine depends on the bearing. While catalog data is sufficient for the majority of applications, it only describes the estimated performance characteristics of bearings. In reality, the performance strays from catalogue stats for reasons that include operating conditions like load, tolerance and mounting configuration. It’s difficult to predict exactly how a bearing will perform until it is assembled and mounted. Testing determines key performance characteristics and whether the bearing can even meet application requirements. Testing makes sense when:
•
The equipment must be reliable
•
In-service repair is extremely difficult or impossible
•
The equipment is expensive
•
The equipment is critical to operations
•
Equipment failure could result in injury or death
•
Certification is required; for example to maintain the warranty.
“At Lancer Systems, we developed a ceramic matrix composite material to replace silicon carbide bearings for fluid film plain bearings,” Braza says. “During the development, it was imperative to generate technical data regarding the performance of the material as fluid film bearing in order to convince our customers to use this material in their pumps. The pump design engineers needed an understanding of the material as a bearing, particularly its pressure velocity limitation, chemical resistance and dryrun capabilities.”
TESTING VS. ISO 281 L10
Bearing tests and standards have been the source of confusion and controversy for decades. Rolling element bearing fatigue life calculations for most industrial and machine applications are dictated in the U.S. by ANSI/ABMA Standard 9 for ball bearings and ANSI/ ABMA Standard 11 for roller bearings.
Outside of the U.S., both ball and roller bearing fatigue life is dictated by ISO Standard 281: 2007, which incorporates a fatigue limit into its
L10 bearing life calculation. It is hotly disputed by bearing experts that include Zaretsky. He and others are certain that no true fatigue limit for a bearing has ever been established and that existing data does not support the establishment of a fatigue limit.
Zaretsky explains, “What can happen when using the ISO Standard 281: 2007 is that the life of a rolling element bearing can be over-predicted for a specific application. Since the bearing life that is predicted is greater than that which may be required, the bearing size is reduced as well as the bearing acquisition cost. This can result in an undersized bearing for the application and early bearing failure. Then there is the matter of warranty and liability issues when the bearing or bearings do not perform as predicted for the application.”
He continues, “It’s important to distinguish between the rolling element bearing’s service life and the bearing’s
L10 fatigue life. Bearing service life can be defined as the time a bearing is removed from service for any cause. The bearing’s theoretical life analysis is based, for the most part, on the
L10 fatigue life. It is my opinion that, with some exceptions, bearing (
L10) fatigue life analysis is no longer theoretical but experimentally established with reasonable engineering and statistical certainty.”
Zaretsky adds that rolling element bearing (service) life, whether based on fatigue or other failure mode, is probabilistic and not deterministic. It is not a calculation of the absolute value of a bearing’s operating time but, rather, the probability that a specific bearing operating under well-defined conditions will equal or exceed a calculated operating time based upon a defined failure mode.
“There is a conundrum associated with bearing fatigue life calculations and bearing service life,” Zaretsky says. “If a bearing is properly designed, installed, lubricated and maintained, it should theoretically fail by classical rolling element fatigue. However, probably less than 5 percent of bearings removed from service are removed because of rolling element fatigue. This means that the probable cause for bearing removal for most applications is not rolling element fatigue (which is the basis for the ANSI/ABMA and ISO standards). The cause for removal and thus the bearing service life depends on the bearing application.”
Zaretsky concludes that ISO 281:2007 does not provide a valid representation of actual bearing life in real- world application conditions.
Nixon adds, “ISO and ABMA standards rely on the assumption that proper bearing quality steels and adequate internal geometric design have been incorporated to give the standard catalog performance-rating placed on the product. But improper internal gegeometry and inadequate steel performance can negate the load rating established by the rating equations.”
IN THE END
“Any company that purchases and uses bearings in their equipment should do adequate testing to validate the bearing supplier’s products,” Nixon says. “It is, however, just as important to validate the supplier’s capability to produce the product to the same specifications on an ongoing basis. Most suppliers can provide acceptable prototype samples but may not be able to maintain specifications in series production. Therefore, scrutiny of the supplier’s quality systems and their manufacturing processes along with supply base is just as important.”
Reliable bearings are essential for many applications, including space development. For example, satellites have a flywheel to maintain them in the correct position and orientation. Some satellites, along with their flywheels’ ultra-high-precision bearings, have been operating seamlessly in space for more than 15 years.
The accuracy of any bearing-equipped machinery is determined by the accuracy of its bearings’ revolution. For example, the deflection from the central axis of a computer’s hard disk drive (which uses ultra-high precision bearings) is less than 100 nanometers (100 billionths of a meter).
This points not only to the necessity for performing tests but choosing the correct test and carefully analyzing the data. While bearing tests have improved markedly during the past 60 years, the challenge to improve bearing life through testing remains.
REFERENCES
1.
Click here.
2.
Per E.V. Zaretsky, more specifically, the
L10 life, in millions of inner-race revolutions, is the theoretical life that 90 percent of a bearing population should reach or exceed without failure at its operating load.
3.
Per E.V. Zaretsky, many engineers don’t understand that the life they are calculating is not based on the point just before no failures will statistically occur but on the point at which 10 percent of the bearings are statistically expected to fail. This mistake can result in warranty and product liability claims for the OEM.
4.
Articulated the book that Zaretsky co-authored and edited,
Life Factors for Rolling Bearings, 2nd Edition, STLE (1997).
5.
Most environmental testing is performed in an A-B comparison format.
6.
Click here.
Jeanna Van Rensselar heads her own communications firm, Smart PR Communications, in Naperville, Ill. You can reach her at jeanna@smartprcommunications.com.