New base oils pose a challenge for solubility and lubricity
Dr. Boris Zhmud & Michel Roegiers | TLT Cover Story July 2009
Key issues with additives must be resolved before severely hydrotreated and hydroisomerized base oils gain widespread acceptance.
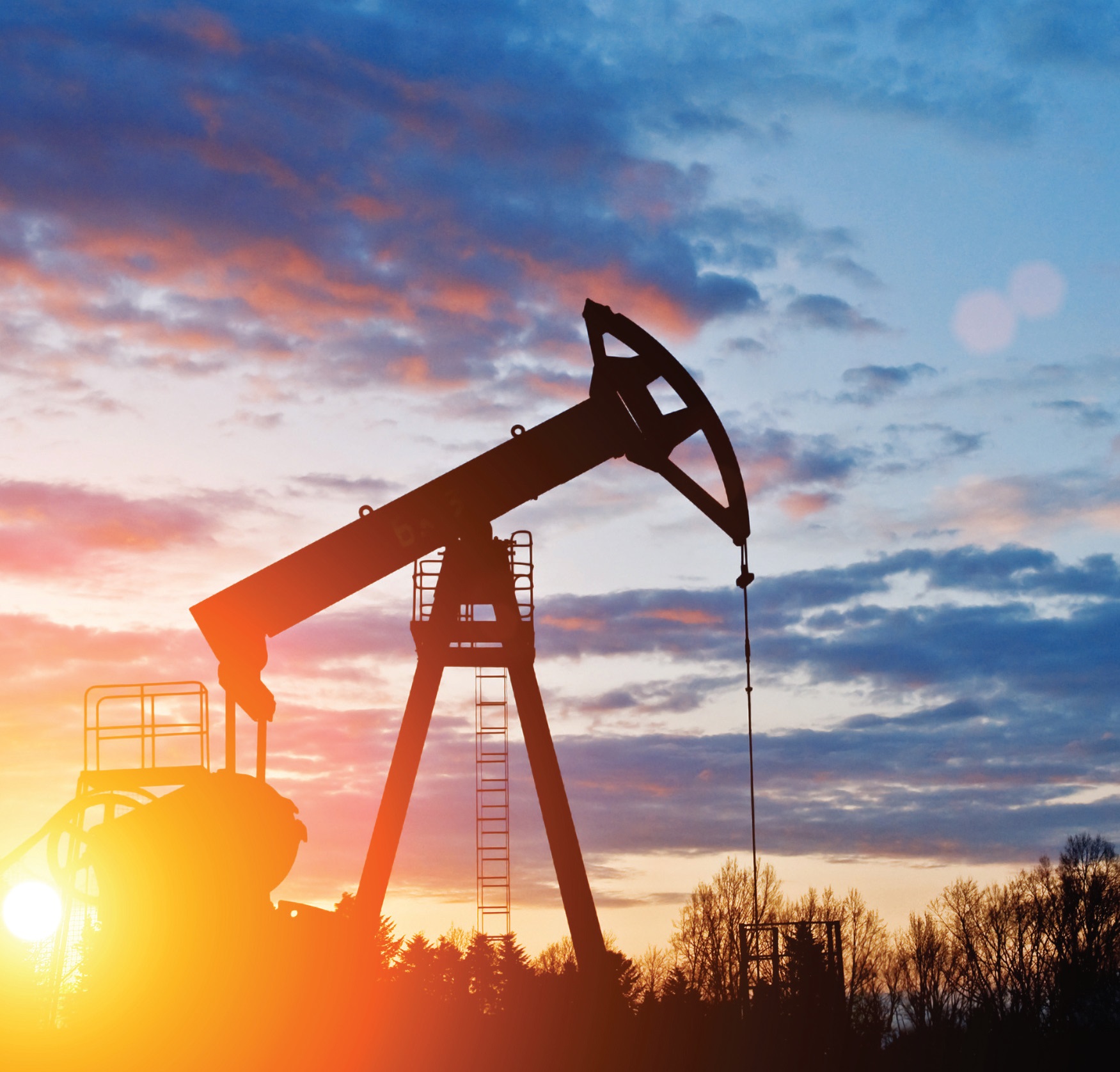
www.canstockphoto.com
KEY CONCEPTS
•
Despite the many undisputed advantages they hold over their Group I counterparts, severely hydrotreated and hydroisomerized base oils have been slow to gain widespread market acceptance.
•
API Group III base oils, as well as GTL basestocks and PAOs, often are regarded as ‘dry’ base oils because they only contain fully saturated non-polar hydrocarbon molecules.
•
The greater the degree of hydrotreatment, the lower the solubility.
Broad commercialization of hydrocracking, catalytic dewaxing and hydrofinishing technologies in the past two decades have created an abundant supply of API Group II and Group III base oils. However, despite the many undisputed advantages over Group I in terms of viscosity index (VI), sulfur content, volatility, pour point and antioxidant response, the new base oils are winning the market much slower than many analysts predicted at the rise of the hydrotreatment technology.
Why is it that difficult? Serious formulators are skillful in property blending, preparing “cocktails” with desired specs being a daily routine for them. There must be something that accounts for formulators’ reluctance to interchange their base oils.
It appears that, when discussing technical implications of the base oil interchange, very little attention is given to solubility and lubricity issues. However, the fact remains that the greater the degree of hydrotreatment, the lower the solubility (
see Scheme 1).
Scheme 1.
Severely hydrotreated base oils, as well as GTL (gas-to-liquid) basestocks and PAO (polyalphaolefins), are often regarded as “dry” base oils because they only contain fully saturated non-polar hydrocarbon (isoparaffin) molecules.
This trend can be easily seen if aniline point values are compared. Lower aniline point means higher solvent power.
For high-aromatic products, such as aromatic extracts, the aniline point is around 20 C-40 C; for naphthenic base oils, 70 C-100 C, depending on the degree of refining and viscosity; for Group I paraffinic base oil, 90 C to 110 C; and for Group II-IV base oils, 100 C-130 C or higher.
It is interesting to note that the aniline point steadily increases with the increasing viscosity for oils with identical polarity. For instance, in the series PAO 2, 4, 5, 6, 8, 10, 40, it raises from ca 100 C for the lightest to ca 160 C for the heaviest homologue. This is because, as can be shown by thermodynamic arguments based on the Hildebrand solubility theory, aniline point depends upon the product V
M(δ
anil-δ
oil)
2 where V
M is the average molecular volume, and δ
anil and δ
oil are the Hildebrand solubility parameters for the aniline and for the oil, respectively. Increasing the average molecular volume raises the aniline point.
Low solubility not only makes it difficult to dissolve some essential additives, it also compromises essential quality parameters such as dispersancy and seal compatibility. For instance, PAOs are unbeatable in terms of pour point and volatility and at the same time have the lowest lubricity and solubility ranking. This is normally compensated by using solubility improvers in finished lubricant formulations.
Theoretically, any chemical compound addition causing a drop in aniline point or an increase in the seal compatibility index in the base oil may be regarded as a solubility improver. However, in practice, many other requirements have to be met such as flash point, pour point, viscosity index, etc. This limits formulators to one of the following strategies:
Blend with naphthenic basestocks or alkylaromates. Improvement in solvency comes at a price of a loss in viscosity index. In contrast to alkylaromates, naphthenic bases have a higher tendency to form sludge, which limits their applications.
Retro-blend with Group I basestocks. Improvements in solvency and lubricity come at a price of deteriorating viscosity index, Noack volatility, pour point, antioxidant response and health safety and environmental (HSE) profile.
Blend with synthetic esters. Improvements in solvency and lubricity come at a price of downgrading stability. Even more dangerous is that some linear-chain esters and their decomposition products passivate the surface against reaction with extreme pressure (EP) additives, undermining antiwear protection at high loads.
Blend with vegetable oils. This technology existed before synthetic esters. Vegetable oils have their own advantages and disadvantages. The chief advantages are the use of a renewable resource, excellent lubricity and antiwear properties, excellent thermal stability, high specific heat, high flash and completely benign HSE profile. The chief disadvantage is low oxidation stability.
Blend with ionized vegetable oils. These products have unique properties, inheriting their positive features such as high lubricity and antiwear efficiency from vegetable oil while at the same time adding oxidation stability and antisludge capability.
With base oils, solvent power is chiefly determined by polarity of oil molecules. PAOs are non-polar, so they have low solvent power. Esters are polar, so they have high solvent power. Alkylated aromatics also have high solvent power, even though they cannot be called polar in the classical sense as the dipole moment of the aromatic rings is close to zero. However, since the system of π-electrons in the aromatic ring is readily polarizable, intermolecular cohesion is sufficiently strong due to induced dipoles. Differences in polarity between various basestocks can be illustrated using the Teas diagram, as shown in Figure 1.
Figure 1. Teas diagram showing relative contributions of various types of intermolecular interactions to the intermolecular cohesive energy. d - dispersion interaction, p - polar interaction, and h – hydrogen-bonding interaction. Group II-IV base oils contain fully saturated hydrocarbon molecules, hence polar and hydrogen-bonding interactions are negligible. Esters reveal more significant polar interactions. Ionized vegetable oils have both polar interactions and hydrogen-bonding interactions.
What does lubricity have to do with all that? Solvent power and lubricity are interrelated properties. This can be proven, both experimentally and theoretically, by studying the adhesion of lubricant films to metal surfaces. Talking about lubricity, one refers to the slipperiness of lubricant films separating the rubbing surfaces from each other. As long as the lubricant film is thick and resilient enough to prevent direct asperity-asperity contact, the coefficient of friction tends to be very low. In this case, one talks about the film lubrication regime.
However, solvent power alone does not guarantee good lubricity. Lubricity requires that polar and non-polar molecules be present simultaneously. Since metal surfaces are highly polar, polar oil molecules dissolved in non-polar ambient tend to adsorb to the metal surface, forming a protective surface film. Strength of the film and solvent power are linked to the same cohesion parameters.
Group I base oils have sufficiently high content of polar species (heterocycles, alkylated aromatics) and demonstrate superior lubricity as compared to Group II-IV base oils. Correspondingly, Group II-IV base oils will benefit the greatest in terms of lubricity from using boundary lubricity additives, also referred to as friction modifiers among tribologists. Many amphiphilic molecules, such as fatty amides, ethanol amides, phosphamides, esters and vegetable oils, can be used as boundary lubricity additives, but performance varies broadly.
Unlike conventional antiwear and extreme pressure additives such as dibenzyldisulfide, tricresylphosphate and zinc dialkyldithiophosphate, which chemically react with metal surfaces when a direct asperity-asperity contact occurs in the boundary lubrication regime, boundary lubricity additives function by physical adsorption onto the rubbing surfaces. In other words, boundary lubricity additives reduce friction and wear by forming adsorbed surface layers (fatty amides, esters) or slippery surface deposits (graphite, teflon), which physically separate the rubbing surfaces from each other, while EP/AW additives reduce friction and wear by chemically reacting to the metal surface under boundary contact conditions to yield a reaction product which prevents cold welding.
It is important to realize that EP/AW additives start to act after the asperity-asperity contact has occurred—but they do not prevent its occurrence. When unlubricated sliding is encountered, friction and material deformation produce the heat needed to trigger the EP/AW reactions. On the contrary, boundary lubricity additives keep their lubricity-enhancing effect, even if there is no reciprocal motion between the rubbing surfaces. That is why they are so efficient in controlling stick-slip and chatter phenomena.
Some tribologists do not differentiate between EP/AW and boundary lubricity additives, referring to both as friction modifiers in general. The reason is that many additives are, indeed, multifunctional, and a reduction of friction usually reduces wear if other conditions are identical (though, there are some exceptions).
For instance, chlorinated paraffins and phosphate esters act as boundary lubricity additives at moderate pressure and temperature, but they start to act as EP/AW additives by reacting with metal surfaces at extreme pressure and high temperatures to yield a surface layer of metal chloride or phosphate. Therefore, it makes more sense to speak about a spectrum of properties associated with each additive in particular rather than trying to classify additives based on selected properties.
One special class of boundary lubricity additives fall outside the existing classification—we call them surface-gelforming friction modifiers or superlubricity additives. Examples are certain amphiphilic ester-based comb-copolymers and Elektrionized™ vegetable oils. These additives form a sponge-like viscoelastic surface layer retaining the base oil in the tribocontact even at zero sliding speed (zero Hersey number), thus expanding the range of operating conditions under which film lubrication is sustained (
see Figure 2).
Figure 2. Stribeck diagram comparing the tribological effect of conventional EP/AW and boundary lubricity additives with the effect of superlubricity (SL) additives forming gel-like surface layers. μ - the coefficient of friction, η - viscosity, v - sliding velocity, and p - applied pressure. High pressure and low sliding velocities force the tribosystem into the boundary lubrication regime in which most intense friction and wear occur. EP/AW additives, as well as regular boundary lubricity additives, shift the Stribeck curve down, reducing friction in the boundary lubrication regime. Superlubricity additives shift the Stribeck curve to the left, maintaining the film lubrication regime over a broader range of tribological conditions.
Superlubricity additives build upon the concept of biomimetic lubrication. Most readers of this article have probably experienced such a superlubricity effect while walking on the slippery rocks of the seashore. What makes those rocks so slippery is the algae slime growing on the rock surface. The algae slime retains a sufficiently thick layer of water between your feet and the rock surface to enable transition from boundary to film lubrication regime under the pressure (equal to your body weight divided by the area of your footstep) when water alone would fail to provide adequate film strength.
It should be realized that commonly used lubricity standards, such as BOCLE (ASTM D 6078) and HFRR (ASTM D 6079), overestimate the effect of EP/AW additives and underestimate the effect of superlubricity additives. This often leads to misunderstandings, terminological muddle and endless debates regarding correlations between laboratory tests and field.
For instance, in HFRR, 200 g load is applied to a 6-mm steel ball reciprocating on a steel flat (AISI52100, 650HV). In this case, the Hertzian contact pressure in the beginning of the experiment is around 1 Gpa, corresponding to a point in the top left end of the Stribeck diagram. Under such a pressure, soft surface gel films produced by superlubricity additives get punctured, and the measured coefficient of friction is dominated by the local coefficient of friction in the high-pressure zone (
see Figure 3).
Figure 3. Pressure map for a steel ball/steel substrate tribocontact in the presence of an adsorbed viscoelastic film produced by a gel-forming friction modifier under the conditions of ASTM D6079 lubricity test. The Hertzian contact pressure in the central point is around 1 GPa, decaying to zero on the periphery of the contact zone. The local coefficient of friction in the 0.1 to 1 GPa pressure zone is ca 0.1 (boundary lubrication), while the local coefficient of friction in the 0 to 10 MPa pressure zone is ca 0.001 (film lubrication). The coefficient of friction measured experimentally is dominated by the greatest term and thus is close to 0.1.
This explains why common EP/AW additives such as molybdenum, phosphate esters and polysulfides always excel in those tests. In other words, standard “lubricity” tests do not really test lubricity—they test the EP/AW functionality. If the rubbing parts in an engine were continuously exposed to such a stress, the engine lifetime would have been limited to a few days! In reality, the majority of tribosystems in cars are exposed to repeated loading-unloading cycles with a typical stress range of 1 to 10 Mpa. The lubricity-enhancing effect of surface-gel-forming friction modifiers is circumscribed to a Hersey number range in which transition from the EHD to the boundary lubrication occurs, and this is outside of the scope of BOCLE and HFRR in their standard setup.
Due to their greatly reduced volatility and good low-temperature performance, new base oils of API Group II-IV allow the formulation of lighter automotive viscosity grade oils such as SAE 5W-40, 0W-30 and even 0W-20 to achieve better fuel economy. However, as explained in Figure 4, the use of thinner base oils increases the risk of engine wear unless appropriate friction modifiers are simultaneously deployed in the formulations.
Figure 4. Relationship between engine oil viscosity, engine wear and fuel economy. Thinner base oils reduce viscous losses but simultaneously increase frictional losses and engine wear. Surface-gel-forming friction modifiers allow one to reduce frictional losses and to maintain good protection against wear while using thinner base oils for better fuel economy.
By shifting the Stribeck curve to the left in Figure 2, friction modifiers cause an equivalent shift of the wear and the frictional losses curves in Figure 4. The result is that the optimal viscosity range (shaded in blue) corresponding to the greatest fuel economy also is shifted to the left toward lower viscosities. In practice, however, it is wise to prefer a somewhat heavier oil to a somewhat lighter one to further minimize wear. After all, changing the oil is more economical than changing the engine!
Changing the oil is more economical than changing the engine!
While solubility and lubricity improvers help formulators address certain challenges brought by a changeover to “dry” basestocks, their use requires some experience and understanding of chemical differences. For instance, the antioxidant response of vegetable-based products improvers is different from that of hydrocarbon bases and, therefore, the antioxidant package may need to be redesigned accordingly. Phosphites and some conjugated dual antioxidant systems such as tocopherol-disulfide are known to be efficient antioxidants for vegetable oils.
In combination with synthetic and severely hydroprocessed VHVI and XHVI mineral base oils, as well as with emerging Group III+, or “Super-Group III” basestocks produced by the Fischer-Tropsch process, lubricity and solubility improvers serve a solid foundation for formulating top-quality lubricants.
Editor’s Note: Previously published in LUBE magazine.
REFERENCES
1.
Mang, T. and Dresel, W. (2001),
Lubricants and Lubrication, Wiley-VCH, Weinheim.
2.
Wang, Y. and Wang, Q.J. (2006), “Development of a Set of Stribeck Curves for Conformal Contacts of Rough Surfaces,”
Tribology Trans.,
49 (4) pp. 526-535.
3.
Zhmud, B. (2007), “Beyond the Aniline Point: Critical Solution Point for the Aniline/Oil System as a Measure of Oil Solubility,”
Fuel,
86, pp. 2545-2550.
4.
Zhmud, B. and Roegiers, M., “Elektrionized Vegetable Oils as Lubricity and Solvency Improvers for Lubricants and Fuels,”
Proc. of the 3rd Asia-Pacific Base Oil Conference, Beijing, China, Sept. 24-26, 2008.
5.
Zhmud, B., “Influence on Lubricant Quality of Base oil Type and Additives,”
Proc. of the 4th International Conference—Lubricants Russia 2008, Moscow, Russia, Nov. 12-13, 2008.
6.
Roegiers, M. and Zhmud, B. (2008), “Tribological Performance of Ionized Vegetable Oils as Lubricity and Fatty Oiliness Additives in Lubricants and Fuels,”
Lube 88, pp. 19-26.
Dr. Boris Zhmud is R&D manager at E-Ion s.a., a manufacturer of vegetable-based lubricant additives. You can reach him at b.zhmud@eion-additives.com.
Michel Roegiers, P. Eng., is the founder of E-Ion and a former regional vice president on STLE’s board of directors. You can reach him at m.roegiers@eion-additives.com.